- 1Institute for Marine and Antarctic Studies, Ecology & Biodiversity, University of Tasmania, Hobart, TAS, Australia
- 2GEOMAR Helmholtz Centre of Ocean Research Kiel, Kiel, Germany
- 3Department of Earth Sciences, University of Oxford, Oxford, United Kingdom
- 4School of Earth and Ocean Sciences, Cardiff University, Cardiff, United Kingdom
- 5Research Centre for Carbon Solutions, School of Engineering and Physical Sciences, Heriot-Watt University, Edinburgh, United Kingdom
Humankind will need to remove hundreds of gigatons of carbon dioxide (CO2) from the atmosphere by the end of the twenty-first century to keep global warming below 2°C within the constraints of the global carbon budget. However, so far it is unclear if and how this could be achieved. A widely recognized idea is to accelerate weathering reactions of minerals that consume CO2 when they dissolve. Acceleration could be realized by pulverizing and distributing gigatons of these minerals onto land (termed “enhanced weathering (EW)”) or sea (termed “ocean alkalinity enhancement (OAE)”) thereby largely increasing their reactive surfaces. However, the desired consumption of atmospheric CO2 during dissolution would inevitably be accompanied by a release of mineral dissolution products (alkalinity, Si, Ca, Mg, Fe, Ni, and maybe others). Here, we approximate their maximum additions to assess potential consequences for pelagic communities (mainly primary producers) and the biogeochemical fluxes they control. Based on this assessment, we tentatively qualify the potential to induce positive and/or negative side effects to be high for Fe, Ni, Si, intermediate for alkalinity, and low for Ca and Mg. However, perturbation potentials are always higher at perturbation hotspots and would be different for EW than for OAE. Furthermore, ecological/biogeochemical consequences of EW/OAE largely depend on the minerals used. We hypothesize that mainly calcifiers would profit in a scheme where CaCO3 derivatives would be used due to beneficial changes in carbonate chemistry. Figuratively, this may turn the blue ocean into a white(r) ocean. When using silicates, the release of additional Si, Fe and Ni could benefit silicifiers and N2-fixers (cyanobacteria) and increase ocean productivity ultimately turning the blue ocean into a green(er) ocean. These considerations call for dedicated research to assess risks and co-benefits of mineral dissolution products on marine and other environments. Indeed, both EW and OAE could become important tools to realize CO2 removal at the planetary scale but associated risks and/or co-benefits should be revealed before deciding on their implementation.
Facing Reality—the Need for Negative Emissions to Meet the <2°C Warming Goal
Anthropogenic emissions of greenhouse gases increase the absorption of heat by the atmosphere thereby inducing global warming (Myhre et al., 2013). It is now widely accepted that anthropogenic warming needs to be limited to not more than 2°C to preserve the Holocene mode of operation of the climate system and thus to prevent intolerable risks for humankind (Schellnhuber et al., 2016). The 2015 Paris Agreement, signed by 194 parties and the European Union, adopted the scientific advice by the Intergovernmental Panel on Climate Change (IPCC) to keep global warming “well below 2°C,” and to “pursue efforts to limit the [average] temperature increase to 1.5°C” (United Nations Framework Convention on Climate Change, 2015). This goal can only be achieved when limiting near future CO2 emissions to ~480–1,130 gigatons (Gt) (Le Quéré et al., 2016; Rogelj et al., 2016). Hence, when continuing at the current emission rate of ~37 Gt CO2 per year, the 2°C emission target would be missed in ~13–31 years from now (Peters, 2016; Rogelj et al., 2016).
The remaining carbon budget to stay below 2°C calls for immediate and profound emission reductions roughly in the range of 1–10% year−1 as anticipated in the techno-economic assessments of the RCP2.6 scenarios (Representative concentration pathways leading to ≤ 2.6 W/m2 additional radiative forcing) (Rogelj et al., 2016; Rockström et al., 2017). What is widely unknown to the public, however, is that even the very optimistic emission trajectories summarized in RCP2.6 largely fail to remain within the carbon budget constraints for 2°C by decarbonization alone (Peters, 2016). In 108 out of the 116 RCP2.6 scenarios assessed in the last IPCC report, this is only achievable because of the large scale implementation of “negative emission technologies (NETs)” which remove CO2 from the atmosphere and store it for geological timescales (Rau et al., 2012; Anderson and Peters, 2016; Peters, 2016; Williamson, 2016; Minx et al., 2018). These NETs must be capable of removing something in the range of 600 Gt CO2 from the atmosphere by the end of the twenty-first century to maintain a likely chance (i.e., >66%) to meet the <2°C goal (van Vuuren et al., 2011; Anderson and Peters, 2016; Fuss et al., 2016; Sanderson et al., 2016). Thus, the inability of societies to initiate dedicated mitigation now, will likely push us toward the future implementation of climate engineering where NETs will likely play a key role.
Could Enhanced Weathering (EW) and Ocean Alkalinity Enhancement (OAE) Help to Solve the Climate Crisis?
Every NET has a range of economical and geospatial limitations. As such, a portfolio of approaches is needed to achieve carbon dioxide removal from the atmosphere (CDR) at the necessary scale (Nemet et al., 2018). Various NETs have been proposed so far but only a few have developed beyond the laboratory. There is yet little public funding, generally no established legal framework, and no social acceptance to ramp up any NET to the necessary scale (Oschlies and Klepper, 2017; Braun et al., 2018; Nemet et al., 2018). The public reservations are justified since NETs raise ethical concerns (Lin, 2013; Lawford-Smith and Currie, 2017; Shue, 2017; Lenzi, 2018) and may be associated with side effects for the Earth system (Keller et al., 2014; Fuss et al., 2018; Gattuso et al., 2018). However, since NETs will very likely become key tools for keeping global warming below 2°C it is vital to assess their associated environmental risks before their initiation (Oschlies and Klepper, 2017).
“Enhanced Weathering (EW)” and “Ocean Alkalinity Enhancement (OAE)” are two related NETs which are among the options to realize CDR (Figure 1). The idea is to accelerate natural rock weathering e.g., by spreading large amounts of pulverized silicate and/or carbonate minerals onto warm and humid land areas (EW) or onto the sea surface (OAE). The increased exposure of these minerals enhances chemical weathering reactions whereby atmospheric CO2 is consumed (Box 1) (Kheshgi, 1995; Schuiling and Krijgsman, 2006). Idealized Earth system model studies have shown that EW and OAE can mitigate climate change significantly when operated at a scale that is appropriate to the challenge (Caldeira and Rau, 2000; Köhler et al., 2010, 2013; Paquay and Zeebe, 2013; González and Ilyina, 2016; Hauck et al., 2016; Taylor et al., 2016; Feng et al., 2017; Lenton et al., 2018).
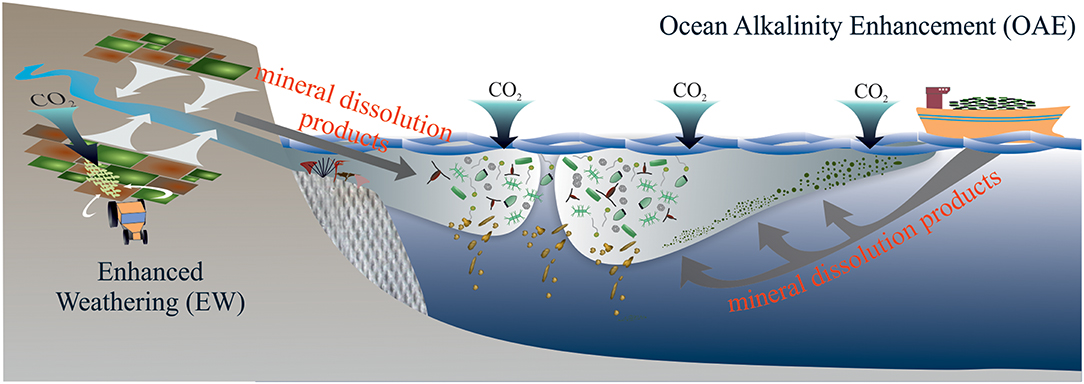
Figure 1. Schematic overview of EW and OAE. EW and OAE will bring mineral dissolution products into marine environments with so far largely unknown consequences for marine ecosystems and biogeochemical cycling therein. During EW, mineral dissolution products enter the ocean mostly via rivers so that perturbations first occur in estuarine and coastal regions. In the case of OAE, cargo ships could transport the minerals to coastal regions or further offshore and the distribution sites would be impacted most strongly.
Box 1. CDR through alkalinity enhancement in aqueous media.
Total alkalinity (TA) is a complex chemical quantity composed of several ions and molecules (Zeebe and Wolf-Gladrow, 2001). TA is defined as the excess of proton acceptors over proton donors with respect to a certain zero level of protons (Dickson, 1981; Wolf-Gladrow et al., 2007). In terms of chemical concentrations this reads as:
The dissolution of CO2 in seawater and the subsequent reaction with H2O have no effect on TA because the formation of proton donors and acceptors is balanced.
A shift of the carbonate chemistry equilibrium (Equation 2) toward and would coincide with decreasing CO2 concentration so that additional CO2 from the environment could be absorbed and stored permanently. Such a shift toward and can be induced through the dissolution of minerals like olivine (Mg2SiO4) or quicklime (CaO). H+ is consumed during the dissolution of these minerals and replaced by conservative ions with positive charges (in our cases Mg2+ or Ca2+) (Pokrovsky and Schott, 2000; Wolf-Gladrow et al., 2007; Oelkers et al., 2018):
The positive charges from Mg2+ and Ca2+ must be balanced by negative ones due to the constraint of electroneutrality (Wolf-Gladrow et al., 2007). This ultimately forces the shift from CO2 to and (Figure 2) and is measurable as an increase in TA which is the name-giving feature of “ocean alkalinity enhancement.”
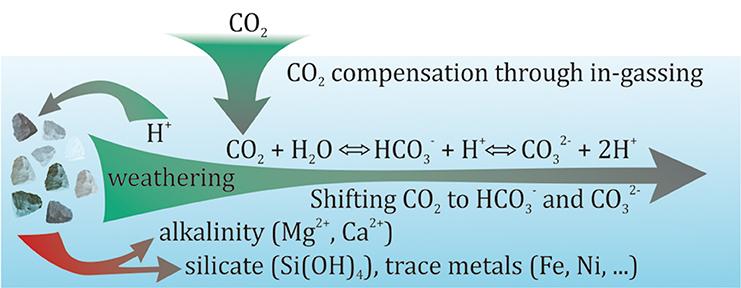
Figure 2. Graphical summary of CDR through chemical weathering in aqueous media (e.g., soil pore water or seawater). When EW/OAE-relevant minerals (e.g., quicklime or olivine) dissolve they consume protons which shifts the carbonate chemistry equilibrium away from CO2 to and . Additional CO2 can subsequently be absorbed by the aqueous media because the shift can cause CO2 undersaturation relative to the surrounding atmosphere. For EW/OAE it is important that the dissolution occurs as long as the aqueous media is in contact with the atmosphere (e.g., in the surface mixed layer of the ocean) so that the under-saturated medium can be replenished with atmospheric CO2.
EW and OAE may be more amenable to implementation (Taylor et al., 2016). First, CDR through rock weathering is a natural process which consumes 1.1 Gt CO2 year−1 already today (Ciais et al., 2013). Second, neither EW nor OAE would require their own land, nutrients, or freshwater (Smith et al., 2016; although dust avoidance during EW may need freshwater, Taylor et al., 2016). They could be applied on open ocean regions or combined with agriculture with the additional benefit of enhancing crop yields and preventing soil erosion (Köhler et al., 2010; Beerling et al., 2018; Dietzen et al., 2018). Thus, in contrast to many other NETs, they are generally not competing with other Sustainable Development Goals like global food and water security but are potentially even beneficial for them (Beerling, 2017; Edwards et al., 2017; Heck et al., 2018). Third, EW/OAE-related alkalinity additions would buffer the CO2-induced decline in seawater pH (Köhler et al., 2010). Thus, EW/OAE would not only mitigate global warming (and its consequences such as sea level rise) by reducing atmospheric CO2 but also specifically mitigate ocean acidification which is considered a major threat for marine ecosystems (Doney et al., 2009; Gattuso et al., 2015).
The minerals appropriate for EW/OAE comprise, for example, naturally occurring Mg-rich olivine-type silicates (Mg2SiO4) (Schuiling and Krijgsman, 2006). These may absorb 1 Gt of CO2 for every 1–2 Gt of olivine-rich rocks when accounting for energy expenses due to mining, mineral grinding, and distribution (Moosdorf et al., 2014). Industrially reprocessed carbonates such as quicklime (CaO) are another option (Kheshgi, 1995). To be effective, the energy-related and the chemical CO2 emissions during the endothermic calcination of CaCO3 in a kiln (i.e., CaCO3 → CaO + CO2) need to be curtailed using carbon capture and storage (CCS) technologies where the generated CO2 is stored e.g., in underground reservoirs. For quicklime, a net removal of 1 Gt CO2 from the atmosphere would require ~1.8 Gt CaCO3 source rock to produce ~0.8 Gt CO2 for CCS and ~1.2 Gt of CaO (Renforth et al., 2013). This estimation includes energy expenses from mining, mineral grinding, and distribution but note that the CO2 emission along the entire process chain could be reduced considerably when using renewable energies (Meier et al., 2006; Renforth et al., 2013).
The natural reserves of source rocks delivering quicklime, olivine, or other suitable minerals are large enough to sequester thousands of gigatons of CO2 (Hartmann et al., 2013; Taylor et al., 2016). The additional mining effort needed to remove several gigatons of CO2 per year roughly equals efforts of the global cement industry which currently extracts ~7 Gt source materials per year (Renforth and Henderson, 2017). Although such amounts are large, they are consistent with the global construction aggregate industry which extracts ~50 Gt year−1 (Beerling et al., 2018). The range of technologies that have been proposed for increasing ocean alkalinity may pose significant engineering challenges. However, preliminary cost analyses suggest that they are within the range of other NETs [$10–500 per ton of CO2 (Strefler et al., 2018; Pacala et al., 2019)]. Thus, if the world is serious about the <2°C goal and anticipated carbon tax revenues are used to subsidize CDR, then EW/OAE could become economically viable options to realize negative emissions in the twenty-first century.
Under these prospects, it is essential that EW/OAE research expands from feasibility and cost studies to include assessments of risks and co-benefits (Oschlies and Klepper, 2017; Gattuso et al., 2018; Boyd and Vivian, 2019). While this is beginning for terrestrial environments (Beerling, 2017; Beerling et al., 2018), very little is known about impacts on marine systems. Here, several side effects have been conceived (Hartmann et al., 2013; Renforth and Henderson, 2017) but only in four cases were these specifically further investigated in silico (Köhler et al., 2013; Hauck et al., 2016) or in vitro (Cripps et al., 2013; Gore et al., 2018).
This paper aims to provide a comprehensive overview on potential risks and co-benefits associated with chemical EW/OAE-perturbations for pelagic environments. The overarching goal is to reveal key knowledge gaps thereby providing guidance for necessary future research.
Chemical Perturbations of Ocean Ecosystems Through EW/OAE
In the case of EW, pulverized minerals would be distributed onto cropland and forests in warm and humid climates (Köhler et al., 2010; Taylor et al., 2016). The dissolution products originating from the chemical weathering reaction would be partially retained in soils and freshwater systems depending on their individual mobilities. However, a certain fraction would ultimately be discharged into the oceans and primarily affect coastal and estuarine systems (Figure 1; Laruelle et al., 2009; Dürr et al., 2011; Gaillardet et al., 2014). Indeed, anthropogenic alkalinity enhancement through agricultural liming and other activities are likely already occurring in various coastal and estuarine systems (Guo et al., 2015; Müller et al., 2016; Kapsenberg et al., 2017; Kaushal et al., 2018) but the impact of this on the organisms and ecosystems is unknown.
In the case of OAE, mineral dissolution products would be directly placed into the surface ocean and influence ocean biota in coastal and offshore regions without previous modification by the “terrestrial filter” (Figure 1). Logistically, minerals would most likely be added at discrete locations because an even distribution over entire ocean regions seems unfeasible (Köhler et al., 2013). This may lead to the formation of “hotspots” of impact, but this will depend on how minerals are added, the type of material added, and the attenuation/mixing in the surface ocean relative to dissolution rate of the mineral. Most likely, they would be (1) concentrated along ship tracks which disperse the material onto the sea surface (Köhler et al., 2013), (2) in the vicinity of on- and offshore platforms from where the dissolution products are released (Rau et al., 2013), or (3) on beaches or shallow shelf seas where minerals could dissolve on the seafloor and would still be within the mixed layer and therefore in contact with the atmosphere (Hangx and Spiers, 2009; Feng et al., 2017; Meysman and Montserrat, 2017). Accordingly, dissolution products from the weathering reaction will occur in gradients and it will be important to reveal how different ecosystems respond to high and low concentrations of mineral dissolution products.
Previous reviews have listed various suitable minerals for EW/OAE (Hartmann et al., 2013; Renforth and Henderson, 2017). The molecules or elements generated during chemical weathering of these minerals and sustained in solution thereafter comprise for major groups: I) Bicarbonate () and carbonate ion (), hereafter summarized as “alkalinity”; (II) Silicic acid (Si(OH)4) hereafter termed silicate; (III) Certain alkaline earth metals like calcium (Ca2+) and magnesium (Mg2+) but potentially also alkali metals like sodium (Na+) and potassium (K+); (IV) a variety of “trace metals” associated with the minerals. Among these, iron (Fe2+, or oxidized aqueous species), nickel (Ni2+) will be the major subject in this paper as these elements occur in high concentrations in basic and ultrabasic rocks (e.g., dunite), which are the most widely recognized source rocks for EW/OAE (Schuiling and Krijgsman, 2006; Hartmann et al., 2013). However, depending on the mineral selected, a wider variety of trace constituents should be considered in the future.
Risks and Co-Benefits of Increased Alkalinity
Perturbation
The desired effect of EW and OAE is to increase the alkalinity in aqueous media so that additional (ideally atmospheric) CO2 can be absorbed (Figure 2; Box 1). Idealized modeling studies suggest that EW/OAE could increase surface seawater alkalinity by about 100 to >2,000 μmol kg−1 by the year 2100 although the upper estimates are based on extreme, likely unrealistic, application scales (Köhler et al., 2010, 2013; Ilyina et al., 2013; Paquay and Zeebe, 2013; Keller et al., 2014; González and Ilyina, 2016; Taylor et al., 2016; Feng et al., 2017; Lenton et al., 2018). Alkalinity itself does not affect biology directly because it is a chemical concept, not an ion or molecule that could be sensed by organisms. Nevertheless, the increase in alkalinity substantially modifies pH and the concentrations of various ions and molecules of e.g., the carbonate system which can directly affect biological processes.
In EW applications, new alkalinity will enter the oceans mostly via rivers or streams. These watercourses are usually oversaturated with CO2 relative to the atmosphere and release globally about 1.8 Gt C year−1 mostly from remineralization of terrestrial biomass (Raymond et al., 2013). Hence, the additional alkalinity from EW would likely have exploited its CO2 uptake capacity before entering the oceans. In the case of OAE, alkalinity is generated in seawater. The perturbation of seawater carbonate chemistry depends on how much alkalinity is added per volume and/or how quickly this volume mixes with surrounding waters. It also critically depends on how fast the perturbed seawater equilibrates with the atmosphere. For example, alkalinity addition would consume CO2 from the surrounding seawater without immediate replenishment from the atmosphere when mineral dissolution occurs in a water body with slow air-sea gas exchange. In such a scenario carbonate chemistry perturbations are more severe than for fast air-sea gas exchange because high pH (i.e., low H+) excursions are not immediately mitigated by in-gassing CO2 (Figure 3). To generate negative emissions with OAE, it must be guaranteed that the generated CO2 deficit in seawater is compensated with atmospheric CO2. Accordingly, carbonate chemistry perturbations will ultimately have to conform with the “air-equilibrated” scenario shown in Figure 3. Nevertheless, reaching this equilibrium may take several years depending on the oceanographic setting (Harvey, 2008; Feng et al., 2017). Thus, more extreme carbonate chemistry perturbations due to “non-equilibrated OAE” at perturbation hotspots may transiently occur (Figure 3) and these hotspots will also have a higher potential to affect marine biota.
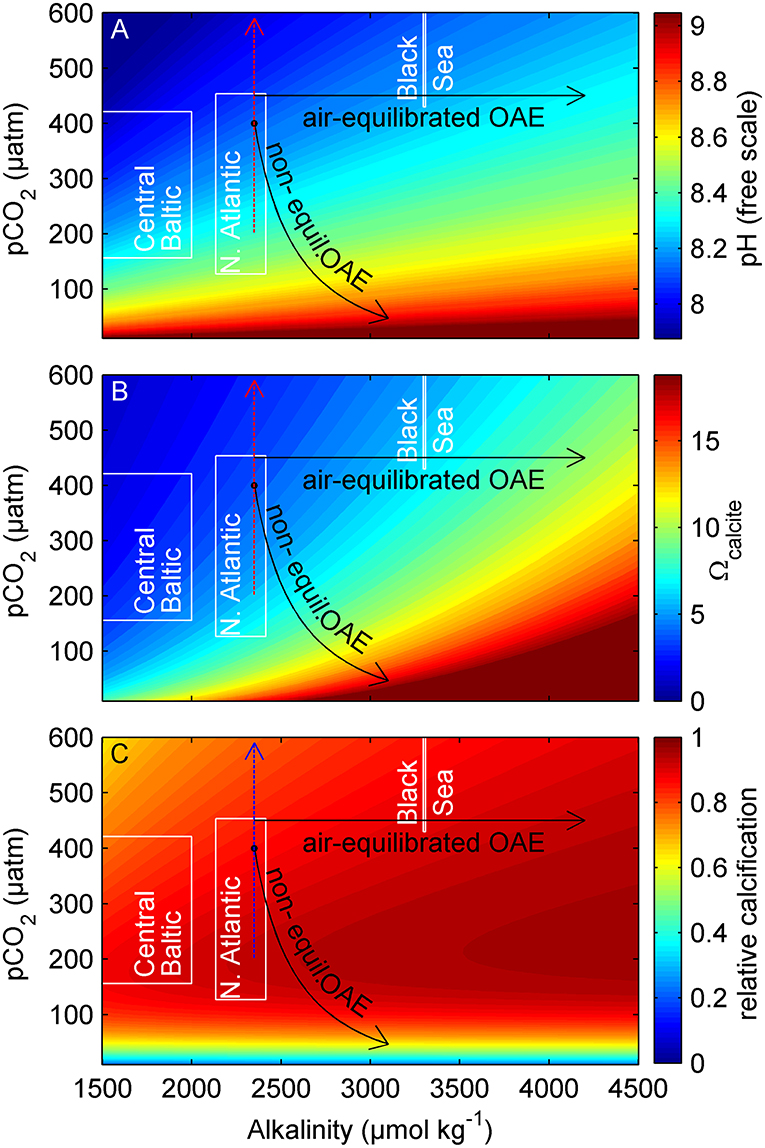
Figure 3. Carbonate chemistry perturbation through EW/OAE. (A) Proton concentration, (B) Ω of the CaCO3 mineral calcite, and (C) relative calcification rates of the coccolithophore Gephyrocapsa oceanica as a function of alkalinity and pCO2. The arrows show the trajectories of ocean acidification (dotted), air-equilibrated OAE, and non-equilibrated OAE. Approximate carbonate chemistry conditions of the Baltic Sea, Black Sea, and North Atlantic (0–100 m depth) are shown in white boxes (data from Goyet et al., 1991; Key et al., 2004; Müller et al., 2016). Calculations were done with CO2SYS (Zeebe and Wolf-Gladrow, 2001) (T = 15° C, S = 35, H+ on free scale, K1, K2 from Lueker et al., 2000). Calcification sensitivity of G. oceanica was determined in experiments and fitted to the coccolithophore calcification model proposed by Bach et al. (2015).
Current Understanding and Key Unknowns
The strong shift in the carbonate system from CO2 to / associated with transient non-equilibrated OAE could potentially drive primary production into CO2 limitation (Riebesell et al., 1993). Phytoplankton, for example, can acclimate to CO2 limitation with carbon concentrating mechanisms (CCMs) which utilize to support C-fixation (Giordano et al., 2005). However, uptake cannot fully compensate for limited CO2 since must ultimately be converted to CO2 for C-fixation and can leak out of the cells before being incorporated in biomass (Rost et al., 2006). The threshold below which phytoplankton growth rates become notably affected by CO2 is genotype-specific and depends on growth conditions (e.g., light or temperature) but should generally be roughly below ~100 μatm (Riebesell et al., 1993; Goldman, 1999; Hansen, 2002; Bach et al., 2011; Sett et al., 2014). Thus, phytoplankton growth could be slowed to a variable degree along gradients of mineral dissolution hotspots. However, dilution with un-perturbed water masses as well as CO2 in-gassing should quickly alleviate the problem so that new production supported by the available nutrients may shift in space and time but rather not with respect to its overall magnitude as it seems unlikely that the nutrients remain unutilized over the course of a seasonal cycle. Nevertheless, the pulses of low CO2/high pH will affect species differentially as some will be more sensitive than others (Hansen, 2002; Pedersen and Hansen, 2003).
Perhaps the most fundamental outcome of more than two decades of ocean acidification research is that carbonate chemistry perturbations affect calcifying organisms disproportionately (Gattuso et al., 2015). Thus, it is meaningful to pay special attention to calcification when considering impacts of increased alkalinity on marine organisms and ecosystems. Ocean acidification (black arrow in Figure 3) is predicted to have adverse effects on calcifiers (Orr et al., 2005; Kroeker et al., 2010), mainly due to the increased proton (H+) concentration and decreased CaCO3 saturation state (Ω) (Jokiel, 2011; Bach, 2015; Cyronak et al., 2015; Waldbusser et al., 2015). EW and OAE have the opposite effect on carbonate chemistry conditions. Here, H+ decreases while Ω increases which could significantly improve the conditions for calcification (Figure 3). The Black Sea is a suitable analog for a highly OAE perturbed marginal sea as it has a surface alkalinity of ~3,300 μmol kg−1 already today (Figure 3). The major planktonic processes in the Black Sea are similar to other oceans (Sorokin, 2002) but there is a remarkable particularity. The Black Sea harbors some of the most extensive blooms of calcifying phytoplankton (coccolithophores) and thick layers of calcareous ooze cover the sediments (Hay, 1988; Kopelevich et al., 2014). These blooms are likely promoted by the favorable carbonate chemistry conditions since coccolithophores are largely absent in the Baltic Sea—a lower alkalinity marginal sea (Müller et al., 2016) with in other respects comparable characteristics to the Black Sea (Tyrrell et al., 2008).
Important Knowledge Gaps and Testable Hypotheses
We have argued in the previous section that transient shifts in carbonate chemistry conditions should have little impact on the overall productivity of marine ecosystems but could alter the species composition. The latter may be important because even though the perturbation is transient, it could induce knock on effects altering species composition and trophic interactions during the succession following the initial perturbation. Such a restructuring could have more severe biogeochemical consequences than initially anticipated. For example, the phytoplankton spring bloom is typically dominated by large phytoplankton species (e.g., diatoms) but in the case of acute OAE perturbations the large species could be replaced by smaller ones which are morphologically better suited to deal with low CO2 concentrations (Wolf-Gladrow and Riebesell, 1997; Flynn et al., 2012; Chrachri et al., 2018). Boyd and Newton (1995) compared organic carbon export to the deep ocean of the 1989 and 1990 North Atlantic spring blooms where a large diatom species was dominant in 1989 and a smaller one in 1990. They found that, while primary production was similar in both years, particulate organic carbon export from the surface to 3,100 m depth was substantially lower in 1990 when the smaller species dominated (Boyd and Newton, 1995). Thus, it will be important to reveal if the transient but possibly pronounced carbonate chemistry perturbations associated with OAE have the potential to alter succession patterns and associated biogeochemical processes such as carbon export on larger scales.
EW/OAE will very likely have a much more sustained impact on calcifiers because the carbonate chemistry changes beneficial for calcification (i.e., increased Ω, ; lower H+) will be permanent (Figure 3). An expected promotion of calcification through EW/OAE will occur at various scales. At first, low H+/ high Ω conditions will be sensed at the physiological level and facilitated CaCO3 precipitation could allow the organisms to divert more energy into other metabolic processes and growth (Jokiel, 2011; Bach, 2015; Monteiro et al., 2016) (b). This may give calcifiers an energetic advantage and could increase their competitiveness relative to non-calcifiers. Indeed, a recent experiment with natural plankton communities demonstrated that differences in seawater pH can cause seemingly small differences in the growth rates of the dominant calcifier (in this case the coccolithophore Emiliania huxleyi) which were sufficient to induce order of magnitude differences in maximum population sizes among treatments (Riebesell et al., 2017). This not only had a considerable influence on the composition of the food web but also strongly altered biogeochemical fluxes such as carbon export or emissions of climate relevant trace gases (Bach et al., 2016; Webb et al., 2016; Riebesell et al., 2017). Accordingly, seemingly small effects of EW/OAE on organism physiology may be amplified in natural environments leading to ecological regime shifts toward calcifying species. Specifying the influence of EW/OAE on pelagic calcification will be important considering the biogeochemical relevance of this process as will be highlighted in the following paragraph.
About 1–2.6 Gt C are precipitated as CaCO3 by marine organisms every year (Lebrato et al., 2010). The vast majority of the CaCO3 is formed by coccolithophores, foraminifera, and pteropods (Sarmiento and Gruber, 2006; Lebrato et al., 2010). The formation of one mole of CaCO3 reduces alkalinity by 2 moles (Zeebe and Wolf-Gladrow, 2001) so that calcification counteracts the desired effect of EW and OAE. For example, net calcification in the surface ocean was increased by 10 % (in mol) through OAE, then 20 % more alkalinity would be consumed and the effectiveness of OAE would be strongly reduced. Furthermore, the EW/OAE-induced increase of Ω could decrease CaCO3 dissolution in sinking aggregates which are CaCO3 under-saturated microenvironments in the surface ocean (Milliman et al., 1999). As a consequence, more alkalinity bound into sinking biogenic CaCO3, would be exported to the deep ocean where its capacity to store atmospheric CO2 is not exploited. These relatively easily quantifiable negative feedbacks are complicated by the influence of pelagic calcification on the efficiency of the biological pump (Armstrong et al., 2002; Francois et al., 2002; Klaas and Archer, 2002). If EW/OAE stimulates calcification, then more CaCO3 ballast material becomes available to accelerate sinking velocities of particulate organic carbon (Honjo et al., 2008; Bach et al., 2016). The accelerated sinking could amplify the efficiency of the biological carbon pump because organic carbon that is built with CO2 originating from the surface ocean would on average sink deeper into the ocean and lock the respired CO2 in the deep ocean for a longer time (Kwon et al., 2009). This amplified deep ocean CO2 sequestration of photosynthetically fixed carbon through the higher availability of ballast CaCO3 could allow CO2 to be taken up more efficiently by the oceans and therefore constitute a positive feedback on the CDR potential of EW/OAE. Thus, the hypothetical proliferation of calcification under EW/OAE applications could have a positive or negative feedback on EW/OAE efficacy, depending on if the “more ballast feedback mechanism” or the “less alkalinity feedback mechanism” become dominant [see (Riebesell et al., 2009) who discussed the influence of these two feedbacks in the context of ocean acidification]. An assessment of the “real” EW/OAE efficacy therefore needs to account for the response of calcifiers to EW/OAE and the biogeochemical feedbacks they drive.
Risks and Co-Benefits of Increased Silicate Concentrations
Perturbation
EW and OAE can be realized with different minerals (Hartmann et al., 2013; Renforth and Henderson, 2017). Olivine-rich silicate rocks like dunite are considered as most effective because they occur naturally, are relatively fast dissolving, and do not require energy-intense chemical processing before their dissolution (Schuiling and Krijgsman, 2006; Renforth and Henderson, 2017). The flipside is that the desired generation of alkalinity coincides with the release of other chemicals such as dissolved silicate (DSi). Dissolving one mole of olivine leads to an increase in total alkalinity by 4 moles and in silicic acid (H4SiO4) by one mole (Köhler et al., 2013; Box 1).
Estimating how much DSi enters pelagic ecosystems is difficult for EW but possible for OAE. The difficulty for EW comes from the unknown fraction of DSi that is retained in soils, as well as on land and in freshwater ecosystems. This retention will most likely be significant although a considerable amount will undoubtedly be discharged into in coastal environments (Köhler et al., 2010; Dürr et al., 2011). OAE inputs are easier to assess because OAE only makes sense when all, or at least the vast majority, of the mineral added to the oceans dissolves in water layers which are in contact with the atmosphere within a few years after dissolution. Therefore, it can be assumed that most of the Si contained in silicates such as olivine would dissolve and enrich the DSi pool in the surface ocean. Assessments under more realistic constraints (e.g., accounting for mineral grinding and distribution) suggest that the maximum scale of mineral addition through OAE lies somewhere at around 10 Gt year−1 which is roughly 70 Tmol DSi year−1 if dunite was used (Box 2). Thus, OAE would be a source of DSi to the surface ocean that 5–14 fold higher than all natural sources combined (Box 2).
Box 2. Additions of key elements in a global OAE scheme.
In this BOX we approximate how much Si, Ca, Mg, Fe, and Ni could be added to the surface ocean under an intense and immediately (i.e., 2020) initiated application of OAE. All approximations should therefore be seen as an upper threshold. Please note that we refrain from approximating element inputs via EW due to the unconstrained degree of element retention by the terrestrial filter. Our estimations are based on idealized scenarios calculated by Köhler et al. (2013), Keller et al. (2014), and Lenton et al. (2018) assuming an even addition of 10 Gt of Mg2SiO4 (Köhler et al., 2013) or Ca(OH)2 (Keller et al., 2014; Lenton et al., 2018) to the surface ocean excluding ice covered areas (Ca(OH)2 “slaked lime” is CaO + H2O). For both minerals this results in a TA addition of around 250 Tmol year−1 because olivine adds double the amount of TA when dissolving but also has roughly double the molecular weight of Ca(OH)2. Lenton et al. (2018) simulated OAE for the twenty-first century also under the low emission scenario RCP2.6 and our estimation follows their scenario because they show that it only makes sense to implement OAE on a global scale when it is accompanied with rapid decarbonization.
The amounts of Si, Ca, Mg, Fe, and Ni added alongside TA strongly depend on the purities of minerals used for OAE. For our estimations we used elemental compositions of dunite provided by the Geochemistry of Rocks of the Oceans and Continents (GEOROCS) database (http://georoc.mpch-mainz.gwdg.de/georoc/). Values given in Table 1 are means ±SD of 609 Si, 604 Ca, 665 Mg, 496 Fe, and 145 Ni dunite samples all of which are globally distributed. For CaO, we used approximate compositions of CaCO3 precursors from America and central Europe (Gabe and Rodella, 1999; Galván-Ruiz et al., 2009; Šiler et al., 2018).
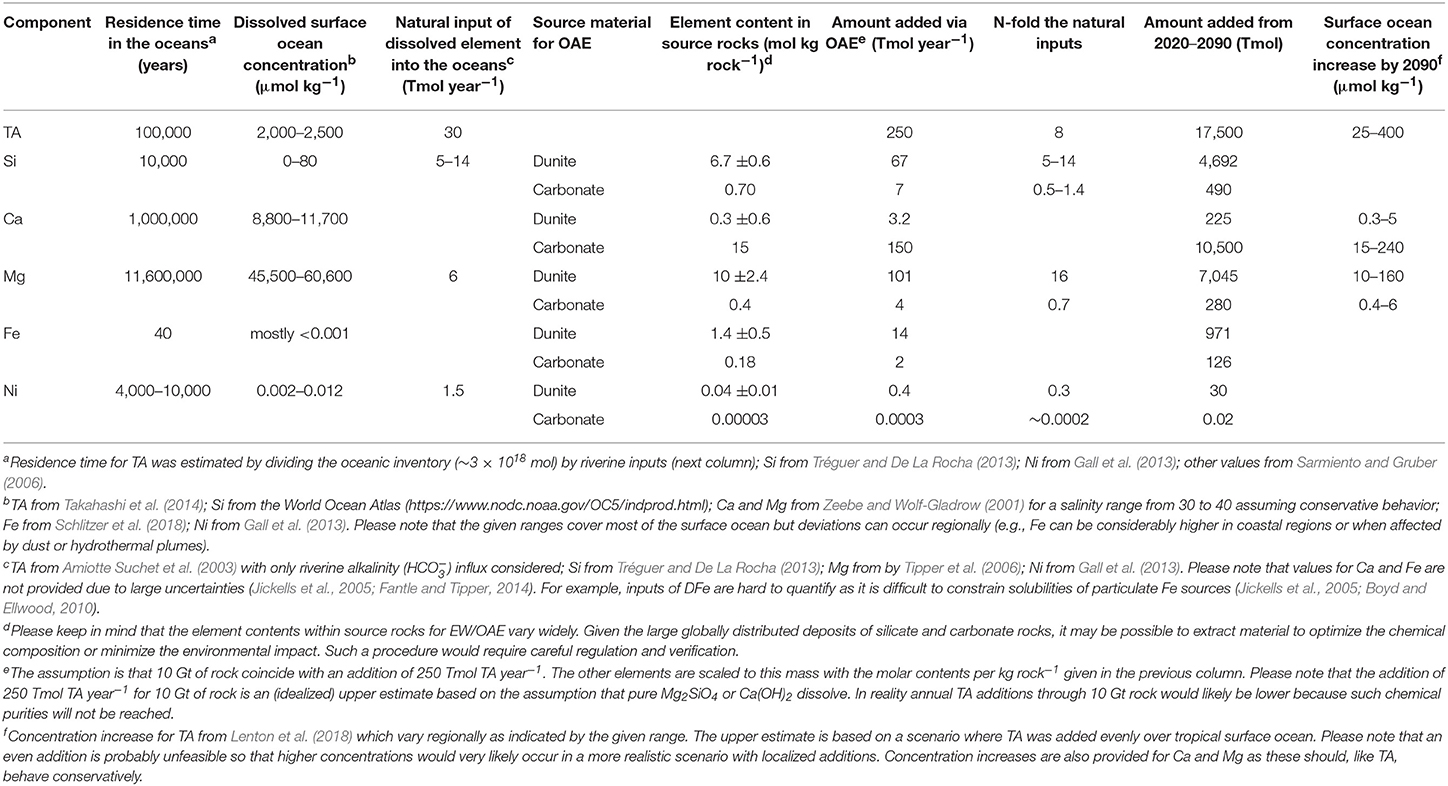
Table 1. Estimated additions of key components in an OAE scenario with 10 Gt rock year−1 (see Box 2 for details).
Table 1 shows that the perturbation potential under an even distribution of minerals scales with element background concentrations in seawater. When considering a relatively even distribution we would consider the perturbation potentials to be high for Si, Fe Ni, intermediate for TA, and low for Ca and Mg. However, as mentioned in the main text, an even distribution of minerals over the entire surface ocean seems unfeasible due to logistic constraints (Köhler et al., 2013). Thus, even those elements marked with a lower potential under these idealized conditions may have significant influence on pelagic ecosystems at perturbation hotspots where they occur at high concentrations.
It is important to emphasize that all values given in Table 1 should be seen as rough approximations. Large uncertainties are, for example, due to large variabilities in chemical source rock composure or uncertainties in estimates of natural inputs of TA and the other elements into the oceans. Furthermore, the added amounts do not account for bioavailability of elements. For example, Si in quicklime could be non-soluble quartz so that inferences on bioavailability must consider solubilities of individual phases. Likewise, Fe has generally a low solubility in seawater (Boyd and Ellwood, 2010) so that a very large fraction of the added Fe would presumably not become bioavailable. Nevertheless, in the particular case of Fe, the amounts that would be added in this 10 Gt dunite-based OAE scenario are so large relative to the surface seawater concentration that they could offset Fe limitation in large parts of the ocean even under the assumption that only 0.1% of dunite Fe becomes bioavailable (Hauck et al., 2016).
Current Understanding
DSi is an essential nutrient for silicifying organisms and by far the most important silicifiers in the ocean are diatoms (Tréguer and De La Rocha, 2013). These are a group of globally distributed phytoplankton that utilize DSi to form a shell (frustule) made of biogenic silica (opal) (Sarthou et al., 2005). Diatoms were estimated to contribute ~25% to primary production on Earth (Nelson et al., 1995; Field et al., 1998). They usually initiate the seasonal phytoplankton succession and form blooms until they become nutrient-limited and other taxa with alternative nutrient acquisition strategies take over (Sommer et al., 2012).
DSi is often the nutrient that constrains diatom proliferation because it is highly deficient relative to other macronutrients throughout most subsurface water masses that supply nutrients to the euphotic zone through upwelling (Sarmiento et al., 2004). In fact it is the very “success” of diatoms throughout the Cenozoic that is thought to have driven the decrease in global ocean Si concentration (Conley et al., 2017). The deficiency in modern subsurface waters likely has two reasons. First, the opaline frustule dissolves relatively slowly so that DSi is remineralized on average at greater depths than other nutrients (Dugdale et al., 1995). Second, much of the subsurface water is formed by the subduction of Southern Ocean surface water (Sloyan and Rintoul, 2001). Before this water mass is subducted and spreads northwards, diatoms strip out and export DSi more than other nutrients thereby trapping DSi in the Southern Ocean (Sarmiento et al., 2004; Primeau et al., 2013; Holzer et al., 2014). These mechanisms explain why global diatom productivity in the present oceans is lower than it could be without prevailing shortage of DSi (Ragueneau et al., 2000; Sarmiento et al., 2004).
Important Knowledge Gaps and Testable Hypotheses
Applications of silicate-based EW and OAE could relieve the present day DSi deficiency (Köhler et al., 2013). The DSi concentrations where diatom DSi uptake rates reach half of the theoretical maximum (K1/2) are species specific and range between 0.2 and 22 μmol kg−1 (Sarthou et al., 2005). High DSi affinity (i.e., low K1/2) typically coincides with lower maximum uptake rates (Vmax). Accordingly, increased DSi in the surface ocean would favor diatoms with high Vmax and K1/2 and therefore presumably fast-growing species (Sarthou et al., 2005). Furthermore, it could allow diatoms to reduce energy investments for DSi acquisition and enable them to construct thicker frustules (Martin-Jézéquel et al., 2000; Sarthou et al., 2005). Energy savings could accelerate diatom growth rates because available energy can be diverted into the acquisition of other nutrients while reinforced frustules would reduce grazing mortality (Hamm et al., 2003; Wilken et al., 2011; Friedrichs et al., 2013; Liu et al., 2016). Indeed, diatoms typically out-compete other phytoplankton when DSi is plentiful and other essential resources are sufficiently available (Armbrust, 2009). Thus, under silicate-based EW/OAE, diatoms could consume a larger fraction of the other limiting nutrients like N and P and ultimately become more dominant than they are already today. However, it will be important to assess if all diatoms profit equally from DSi enrichment due to the large range of DSi affinities among species (Sarthou et al., 2005). In theory, more heavily silicified diatom species should profit over proportionally from silicate-based EW/OAE as these have inherently higher DSi requirements to satisfy. Thus, it will be important to unravel if silicate-based EW/OAE has the potential to shift diatom communities toward more heavily silicified species.
A particularly interesting aspect in the context of community shifts is the competition between calcifiers and silicifiers. We hypothesized above that calcifiers profit from alkalinity enhancement and would become more dominant under EW/OAE. However, a prerequisite for the success of calcifiers would be that growth of the usually more competitive silicifiers is kept in check by DSi limitation (Egge and Jacobsen, 1997; Tyrrell and Merico, 2004). Under silicate-based EW/OAE, this limitation is relieved so that the fertilization of calcifiers through alkalinity enhancement could be overcompensated by DSi fertilization on silicifiers. Humborg et al. (1997) provide an excellent case study where this effect may actually have been observed. The river Danube contributes ~70 % of the river inputs into the Black Sea but was dammed in the early 1970s. The damming reduced DSi inputs into the Black Sea and promoted coccolithophores at the expense of diatoms (Humborg et al., 1997). The reverse case (i.e., increase of DSi) would be true for silicate-based EW/OAE. Here, calcifiers may not be able to benefit from more favorable carbonate chemistry because silicifiers benefit at the same time. Addressing this is very important because the balance between calcification and silicification has tremendous influence on the efficiency of EW/OAE. For example, if DSi inputs reduce net calcification and the subsequent export of CaCO3 into the deep ocean by 10 % (in mol), then 20% more alkalinity remains in the surface ocean to absorb atmospheric CO2. Such a reduction of marine alkalinity sink would enhance the CO2 uptake capacity of the oceans and therefore increase the effectiveness of EW/OAE. Indeed, paleo-oceanographic studies suggest that increased DSi leakage from the Southern Ocean during glacial periods may have led to a shift from calcification to silicification globally (Brzezinski et al., 2002). This could have reduced the marine alkalinity sink and enhanced the oceanic CO2 uptake capacity to such an extent that it can explain parts of the glacial-interglacial difference in atmospheric pCO2 (Matsumoto et al., 2002). It may therefore be an argument to use silicate minerals for EW/OAE as this may reduce the biotic alkalinity sink and thereby indirectly increase the EW/OAE efficacy.
A shift from calcification to silicification under silicate-based EW/OAE would also alter quality and quantity of ballast material in water column. CaCO3 has a higher density (2.7 g cm−3) than BSi (2.1 g cm−3) and is therefore considered to be more effectively accelerating the sinking of organic matter into the deep ocean (Klaas and Archer, 2002). However, CaCO3 ballast is currently considered to be only regionally important in the oceans whereas the influence of BSi ballast is thought to be more widespread (Wilson et al., 2012; Le Moigne et al., 2014; Tréguer et al., 2018). Accordingly, a further increase in diatom biomass and potential shifts toward more heavily silicified diatoms at elevated DSi inputs (see above) may amplify the ballast potential of BSi and therefore carbon export in silicate-based EW/OAE schemes (Köhler et al., 2013). Thus, we speculate that the proliferation of silicifiers, even if this comes at the expanse of less pelagic calcifiers, could lead to a net increase of CO2 sequestration into the deep ocean and therefore constitute a positive feedback on EW/OAE efficacy. If true, this could be another argument for silicate minerals.
Risks and Co-Benefits of Increased (Earth) Alkaline Metal Concentrations
Perturbation
It is expected that the most likely minerals to be used for EW/OAE are Ca or Mg oxides (e.g., CaO) and/or Ca or Mg silicates (e.g., Mg2SiO4). Ca and Mg are important ions for all organisms and in particular for calcifiers: Ca as a main ingredient and Mg as an inhibitor of precipitation which is excluded from the mineralisation space (Davis et al., 2000). Due to the high background concentrations of these alkali Earth metals in seawater, the relative change in their concentrations through addition to the surface ocean will be small (Box 2). However, it is likely that there will be considerable local perturbations through EW/OAE, and therefore Ca and Mg, at the input location for these schemes, on a regional scale. Biotic impacts through Ca or Mg may be pronounced at these perturbation hotspots before mixing through ocean currents disperses these chemicals to lower concentrations.
Current Understanding
High intracellular calcium is incompatible with life. At high intracellular concentrations, Ca2+ causes aggregation of proteins and nucleic acids, affects the integrity of lipid membranes, and can precipitate with cytosolic phosphate removing a major nutrient from bioavailability within the cell (Case et al., 2007). All forms of life, from their origin, need an effective system for Ca homeostasis, which keeps intracellular Ca2+ at concentrations low enough to prevent apatite precipitation, at levels of ~0.1 μM, and ~10,000–20,000 times lower than that in seawater (Carafoli, 1987). Although maintenance of such a large Ca2+ concentration gradient can be used effectively for Ca signaling as a core component of cellular regulation, it comes at a metabolic cost. Thus, any perturbation of the environmental Ca2+ concentration could impose an additional metabolic cost to all forms of life from bacteria to the highest eukaryotes, meaning that marine food webs could be affected by high Ca2+ at perturbation hotspots.
Magnesium is the second most abundant cellular cation after potassium. In comparison to Ca, high intracellular concentrations of total and free magnesium ion (Mg2+) appear to be essential to regulate numerous cellular functions and enzymes, including ion channels, metabolic cycles, and signaling pathways up with intracellular concentrations ranging from 17-20 mmol kg−1, just 2.5 times lower than ambient seawater (Romani, 2011). Understanding how cells regulate Mg2+ homeostasis remains incomplete but again must occur at some, but lesser than Ca, energetic cost to the cell (Romani, 2011).
Important Knowledge Gaps and Testable Hypotheses
From a biomineralization perspective, it is possible that calcification will benefit from additional Ca2+ in a quicklime OAE scheme (Stanley et al., 2005). The influence of Mg in an olivine scheme is more complicated. Mg is known to be a strong inhibitor of inorganic calcite precipitation through incorporation into the CaCO3 lattice (Davis et al., 2000). Mg incorporation raises the dissolution rate of the advancing crystal edge, which subsequently increases the mineral solubility, resulting in corresponding reduced net calcification. Aragonite is not inhibited by Mg incorporation into the CaCO3 lattice in the same way that calcite is. Incorporation of Mg into aragonite's crystal structure has been shown experimentally to have no effect on growth rate of aragonite crystals (Berner, 1975; Gutjahr et al., 1996). Changes in the concentration of Mg in seawater relative to Ca do appear to be reflected in the plankton fossil record. Here, high Mg/Ca ratios through low absolute Ca concentrations, as in the modern ocean, promoted aragonite, and high Mg-calcite mineralizers. Conversely, low Mg-calcite mineralizers such as the coccolithophores, were more prevalent during the Mesozoic, which was characterized by oceans with low Mg/Ca and high absolute Ca2+ concentrations (Stanley and Hardie, 1998; Ries, 2010). Therefore, studies in palaeoceanography may be useful to elucidate future changes we could expect from EW/OAE scenarios by acting as suitable analogs.
There is also the possibility that higher Ca2+ and Mg2+ concentrations may interfere with the uptake of nutrients by phytoplankton (and/or bacteria). For example, Ca and Mg have been shown to influence Fe uptake in the freshwater cyanobacteria Microcystis aeruginosa (Fujii et al., 2015). It is therefore possible that Ca and Mg have similar effects on marine phytoplankton which may have implications from primary production at EW/OAE perturbation hotspots.
Given that foraminifera mineralize first by the engulfing of seawater by a membrane, the low Mg/Ca of their calcite compared to inorganic predictions suggests an active role for pumping of Mg away from the region of mineralization (Segev and Erez, 2006). Two species of benthic foraminifera incorporate more Mg into shells as Mg/Ca increases, suggestive that control on the Mg content of the mineralizing space is not strictly regulated. The greatest increase in precipitation of CaCO3 was obtained at Mg/Ca of 1, and not at present-day seawater ratio (Mg/Ca = 5). Thus, it is possible that any decrease (increase) in the Mg/Ca ratio of the ocean will both increase (decrease) the degree of calcification of foraminifera, in addition to making the carbonate less (more) soluble by lowering its Mg/Ca ratio.
Pteropods are holoplanktonic gastropod molluscs which are the major producers of aragonite in the surface ocean today (Andersson et al., 2008). The response of pteropods to changing Mg/Ca is not well-constrained due to the difficulty of keeping pteropods alive in culture (Howes et al., 2014). The origination of the pteropods and rise in prevalence during high Mg/Ca in the Cenozoic may suggest that pteropods are favored by the emerging aragonite sea conditions (Burridge et al., 2017). Accordingly, they could be influenced oppositely to coccolithophores and foraminifera by Mg/Ca alterations associated with EW/OAE.
As a first step, it will be essential to constrain the impact of elevated Ca and/or Mg on the order of magnitude predicted in Table 1. To our knowledge, no work has specifically been completed on the response of plankton other than coccolithophores or foraminifera to increased Mg, Ca or altered Mg/Ca. This work may also be important to complete for the major group of silicifiers, the diatoms, due to the competition between silicifiers and calcifiers mentioned before. If CaO were added to the ocean, thereby decreasing Mg/Ca, it is reasonable to hypothesize that coccolithophores as competitors of diatoms would be further strengthened and take a larger share of the available nutrient pool. There is huge opportunity for the utilization of palaeoceanographic studies to help with these predictions, due to the rich fossil and sediment record providing evidence of secular changes in Mg/Ca ratios over geological time showing that silicifiers have risen to open ocean dominance in high Mg/Ca oceans (Falkowski et al., 2004).
Risks and Co-Benefits of Increased Trace Metal Concentrations
Perturbation
All minerals suitable for EW/OAE will contain elements considered as “trace metals” (Hartmann et al., 2013; Schlitzer et al., 2018). Trace metals occur at relatively low concentrations in seawater so that perturbations at the scale anticipated for EW/OAE are likely sufficient to have a fertilizing and/or toxic effect on organisms (Hartmann et al., 2013; Moore et al., 2013; Hauck et al., 2016). The diversity of trace metals added to the oceans via mineral dissolution would be large and highly dependent on the source mineral used for EW/OAE. For example, alkaline industrial residuals such as steel slags are considered to be useful for EW/OAE but they can contain trace metals such as Cr, Mo, Ni, Pb which could negatively influence the environment above critical threshold concentrations (Mayes et al., 2008; Renforth, 2019). Likewise, naturally occurring minerals like olivine or source CaCO3 for the production of CaO are associated with various elements, depending on their source region. It is beyond the scope of this paper to discuss the potential impacts of all trace elements contained in source material appropriately. We therefore focus on two particularly important ones—iron (Fe) and nickel (Ni). Fe is in focus because it is one of the key nutrients influencing pelagic ecosystems in the surface ocean (Moore et al., 2013; Tagliabue et al., 2017) and is abundant in minerals considered for EW/OAE (Hartmann et al., 2013). Ni is currently not considered as a key nutrient in the surface ocean (Moore et al., 2013) but its abundance in dunite, one of the major candidate rocks for EW/OAE, is very high so that highlighting this element is very reasonable (Meysman and Montserrat, 2017; Beerling et al., 2018).
Fe is rather immobile in soils (Gaillardet et al., 2014) so that Fe enrichment of rivers through EW should be relatively small. However, differences exist among rivers with those that contain large amounts of dissolved organic ligands (“blackwater rivers”) likely transporting more dissolved Fe to the oceans (Krachler et al., 2015; Vieira et al., submitted) which is an important aspect to consider when selecting EW application sites on land. Once riverine Fe reaches the ocean, much of it will be rapidly scavenged, for example by salt-induced flocculation or by settling particles so that Fe perturbations through EW would be restricted mostly to coastal regions (Boyd and Ellwood, 2010) even though physical conditions at some estuaries (e.g., Congo) could transport material further offshore (Vieira et al., submitted). Ni is not as abundant as Fe in olivine but is more mobile in soils and more soluble in seawater (Gaillardet et al., 2014; Montserrat et al., 2017) so that Ni inputs through EW could be higher in coastal systems and potentially further off-shore. OAE short-circuits the terrestrial filter so that the trace metal contamination of the ocean realm would be significantly larger and directly depend on the dissolution rates of mineral powder and the solubilities of the dissolution products (Montserrat et al., 2017).
Current Understanding (Fe)
Fe is an essential micronutrient needed as a co-factor in many enzymes (Tagliabue et al., 2017). The concentration of dissolved Fe (DFe) is mostly <1 nmol L−1 in the surface ocean where productivity is in large parts Fe-limited (Moore et al., 2013; Schlitzer et al., 2018). Therefore, even a relatively small Fe addition of a few tons to Fe-limited surface ocean regions can induce a massive phytoplankton bloom visible from space (Boyd et al., 2007). The ideal olivine mineral for EW/OAE would be pure forsterite (Mg2SiO4; Box 1) but in nature such purity rarely exists. Here, olivines typically occur as a mixture of forsterite and fayalite (Fe2SiO4) (Taylor et al., 2016). A commonly found forsterite/fayalite mixing ratio is ~9:1 and this rock type, termed “dunite,” exists in sufficiently large quantities to sequester maybe some hundreds of Gt CO2 from the atmosphere (Taylor et al., 2016). Fe enrichment scenarios tested with an Earth System model suggested that this iron fertilization of ocean primary production would increase OAE efficacy roughly by one third (Hauck et al., 2016). However, more recent thermodynamic modeling indicated that fayalite dissolution reduces alkalinity through secondary reactions (Griffioen, 2017), although this could not be confirmed in a dissolution experiment (Montserrat et al., 2017). Thus, the overall influence of Fe perturbations on the CDR potential of EW/OAE is very difficult to determine with our current level of understanding. Fe enrichment could, to a smaller extent, also occur under applications of artificially processed minerals. Quicklime for example is made by calcining natural CaCO3 rich source rocks with purities of <98 % typically achieved at industrial scale (Renforth and Kruger, 2013; Renforth et al., 2013). The majority of the remaining mass is composed of silicate and iron oxides. Therefore, Fe or Si addition when using calcined limestone will certainly be lower than from silicates such as dunite (Box 2).
Important Knowledge Gaps and Testable Hypotheses (Fe)
Iron is often not the most limiting nutrient for phytoplankton in coastal environments as these usually receive DFe supply from sediment fluxes or terrestrial sources (Boyd and Ellwood, 2010). Increased DFe river discharge through EW may therefore have a limited effect on primary production near coasts. In the case of OAE the situation could be different. Counterintuitively, the addition of mineral particles could scavenge bioavailable DFe thereby reducing primary production. This may apply for mineral powder additions in DFe replete regions where DFe scavenging (adsorption) by volcanic ash particles has been shown to outweigh the Fe release through dissolution thereby leading to a net removal of DFe (Rogan et al., 2016). However, when particles are added to a DFe deficient system the dissolution term outweighs the scavenging term leading to a net increase in DFe (Rogan et al., 2016). Thus, OAE applied in DFe-limited areas should enhance primary production unless the ecosystem is acutely co-limited by other nutrients such as nitrate. Indeed, DFe and N co-limitation is widespread, especially on the edges of sub-tropical gyres (Browning et al., 2017). OAE would strongly relieve DFe but not N limitation thereby favoring N2 fixing cyanobacteria (e.g., Trichodesmium) which are largely controlled by DFe availability in oligotrophic systems (Mills et al., 2004). It will therefore be essential to test if OAE could stimulate N2 fixation rates of cyanobacteria in these regions that may ultimately increase primary production and the CDR efficacy of OAE.
Altogether, the DFe related effects on primary production would most likely coincide with pronounced shifts in the composition of the plankton community. For example, a specific stimulation of cyanobacteria may initiate a reorganization of the energy flow along trophic pathways, ultimately affecting the marine food web in its entirety. Such indirect effects of EW/OAE may even be stronger than the first order chemical perturbation and therefore need to be taken into account (Strauss, 1991; Walsh, 2013). Ultimately, all the positive and negative side-effects that have been anticipated for ocean fertilization with iron (e.g., increased ocean productivity, deep-ocean hypoxia; Oschlies et al., 2010) would also have to be considered in the context of EW/OAE when minerals with considerable Fe content are used.
Current Understanding (Ni)
Ni is rare in the Earth's crust but over-proportionally abundant in forsterite-rich olivine (Simkin and Smith, 1970). Ni is more mobile in soils than Fe especially at low pH and dissolves relatively quickly so that it is currently considered as the most important contaminant under silicate-based EW/OAE (Nieminen et al., 2007; Hartmann et al., 2013; Meysman and Montserrat, 2017; Montserrat et al., 2017; Beerling et al., 2018). It is an essential co-factor in variety of enzymes but Ni nutrient requirements and sensitivities to excess concentrations vary greatly among organisms (Figure 4). Eukaryotic microalgae have lower Ni requirements (mostly <2 nmol kg−1) and are more sensitive to high concentrations than prokaryotes such as cyanobacteria (Figure 4). Ni has a higher solubility than Fe and occurs in the surface ocean mostly as Ni2+ in concentrations of about 2–12 nmol kg−1 so that bulk primary production is usually not limited by it (Konhauser et al., 2009; Gall et al., 2013; Moore et al., 2013). This also explains the general absence of a nutrient-like vertical concentration profile of Ni with the exception of the North Pacific (Glass and Dupont, 2017) where occasional Ni limitation of cyanobacteria communities have been observed (Dupont et al., 2010).
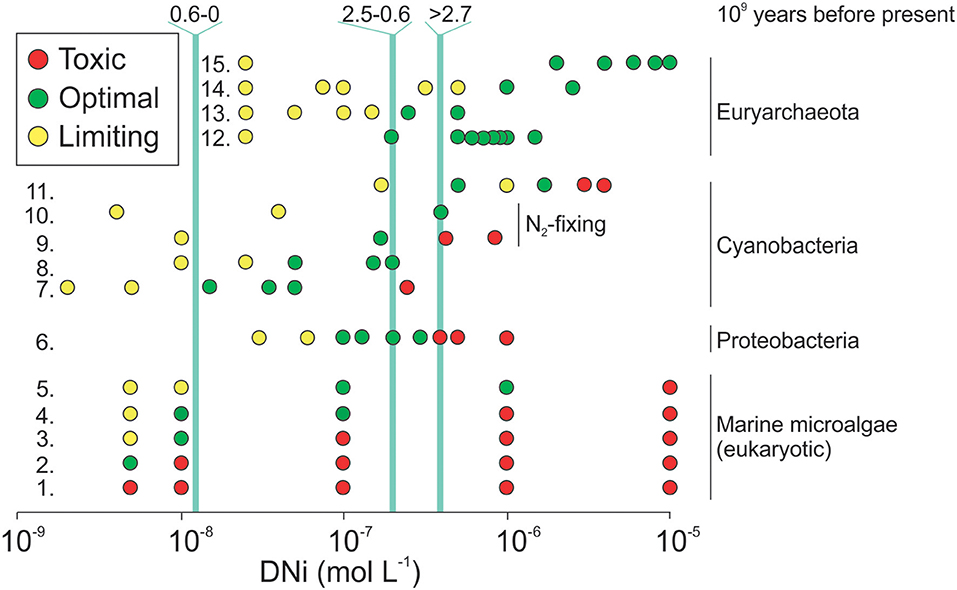
Figure 4. Effects of DNi on growth of (marine) microbes. Marine microalgae were grown in urea. The phyla of each microalgae is given in brackets behind each species name based on www.AlgaeBase.org (Guiry et al., 2014): 1. Pavlova lutheri (Haptophyta), Chaetoceros gracilis (Bacillariophyta “diatom”), Olisthodiscus luteus (Ochrophyta); 2. Prymnesium parvum (Haptophyta); 3. Rhodomonas sp. (Cryptophyta), Achnanthes brevipes (Bacillariophyta “diatom”), Amphidinium carterae (Miozoa “dinoflagellate”), Skeletonema costatum (Bacillariophyta “diatom”), Hymenomonas elongata (Haptophyta), Porphyridium cruentum (Rhodophyta); 4. Thalassiosira spp. (Bacillariophyta “diatom”); 5. Cyclotella cryptica (Bacillariophyta “diatom”); Proteobacteria: 6. Cupriavidus necator; Cyanobacteria: 7. Synechococcus spp.; 8. Oscillatoria sp.; 9. Nostoc muscorum (freshwater); 10. Trichodesmium erythraeum; 11. Arthrospira maxima; Euryarchaeota: 12. Methanococcus voltae; 13. Methanococcoides methylutens; 14. Methanobacterium thermoautotrophicum; 15. Methanothrix soehngenii; Light blue lines are reconstructed paleo seawater DNi concentrations in 109 years before present. The figure was adopted from Glass and Dupont (2017) who compiled the data from numerous references. Reproduced by permission of The Royal Society of Chemistry.
Important Knowledge Gaps and Testable Hypotheses (Ni)
The influence of dissolved Ni (DNi) on pelagic systems has not been a particularly strong focus in Biological Oceanography so far but it may become important in the context of EW/OAE. Here, DNi could either function as nutrient or toxin with pronounced impacts on primary production and plankton community composition.
Recent dissolution experiments with olivine have shown that an increase of alkalinity of about 100 μmol L−1 coincided with an increase of ~3 μmol L−1 in dissolved Ni (DNi) due to non-stoichiometric dissolution (Montserrat et al., 2017). These DNi concentrations are ~3 orders of magnitude higher than ambient concentrations and would likely be harmful for many organisms (Figure 4). Thus, olivine-based EW/OAE could strongly affect marine plankton communities near perturbation hotspots. As for Fe, impacts of DNi will likely differ regionally and depend on the constitution and evolutionary history of plankton communities. For example, communities near estuaries may be adapted to higher DNi concentrations, especially when discharging rivers come from basaltic, olivine rich catchment areas (Nieminen et al., 2007). However, this should be the exception rather than the rule so that we would expect rather detrimental effects on biodiversity and productivity when too much DNi is released locally.
DNi is generally not considered to be an element that is bio-accumulated strongly by organisms (bio-accumulation = enrichment within organism tissues) (Nieminen et al., 2007). Without bio-accumulation, there is also little potential for Ni bio-magnification along the food chain (i.e., Ni enrichment with increasing trophic level). However, as mentioned earlier, Ni is likely one of the most important trace metal contaminants under olivine-based EW/OAE but certainly not the only one. Other potential trace metals (e.g., Cr, Cu, or Cd) (Simkin and Smith, 1970; Beerling et al., 2018) may become bio-accumulated and bio-magnified, particularly in phytoplankton evolutionarily associated to the “red lineage” (Wilson et al., 2019). Furthermore, a suite of anthropogenic materials (e.g., slag from the production of steel, or mining residuals) could be used for EW (Renforth, 2019). These can contain a range of elemental compositions, and a material by material assessment is required for wider-scale impact. Thus, it may be possible that certain trace metals introduced into the environment via EW/OAE could bio-accumulate and bio-magnify which may cause health problems, particularly for end-members in the food chain like humans (Järup, 2003). It is therefore important to identify minerals associated with such risks and not use them for EW/OAE. Indeed, trace metal toxicity may become a key decisive factor for the acceptability of EW/OAE applications and strongly influence the choice of rocks that could ultimately be used.
The toxicity-related hypothesis outlined above refers to EW/OAE scenarios where very high DNi concentrations occur. However, after dilution of the perturbation signal in the oceans through space and time DNi may dilute from harmful to fertilizing concentrations (Figure 4). Ni is an essential co-factor for enzymes involved in urea cycling and utilization (Nieminen et al., 2007; Glass and Dupont, 2017) and has been shown to limit growth of cyanobacteria- and picoeukaryota-dominated phytoplankton communities (Dupont et al., 2010). Therefore, EW/OAE-related DNi inputs could stimulate primary production in certain oligotrophic settings. Indeed, the growth rate of the predominant N2-fixer Trichodesmium has been shown to profit strongly from DNi amendments (Ho, 2013) such that ecological regime shifts toward more cyanobacteria in subtropical gyres would be a plausible consequence of olivine-based EW/OAE. If true, moderate increases of DNi may under certain circumstances locally enhance marine productivity and therefore EW/OAE efficacy through a stimulation of N2-fixation.
Synthesizing the Influence of Different Perturbation Schemes in a Common Framework
In the sections above we highlighted potential risks and co-benefits associated with individual mineral dissolution products. In the following we try to synthesize the various factors in a common framework to establish potential scenarios under sustained EW/OAE.
EW and OAE at the scale needed for relevant CDR could shift the present day ecological/biogeochemical equilibrium (“blue ocean”) into a new steady state depending on the applied source mineral (Figure 5A). Quicklime or other industrially reprocessed minerals should contain relatively fewer contaminants (Box 2) so that EW/OAE would mostly constitute an increase of alkalinity and Ca2+. Here, calcifiers should profit from the favorable carbonate chemistry while silicifiers and N2-fixers are affected to a minor extent (perhaps slightly negatively because calcifiers acquire more of the available nutrients). In such a scenario, the blue ocean could turn into the “white(r) ocean” where calcifiers become more important (Figure 5B).
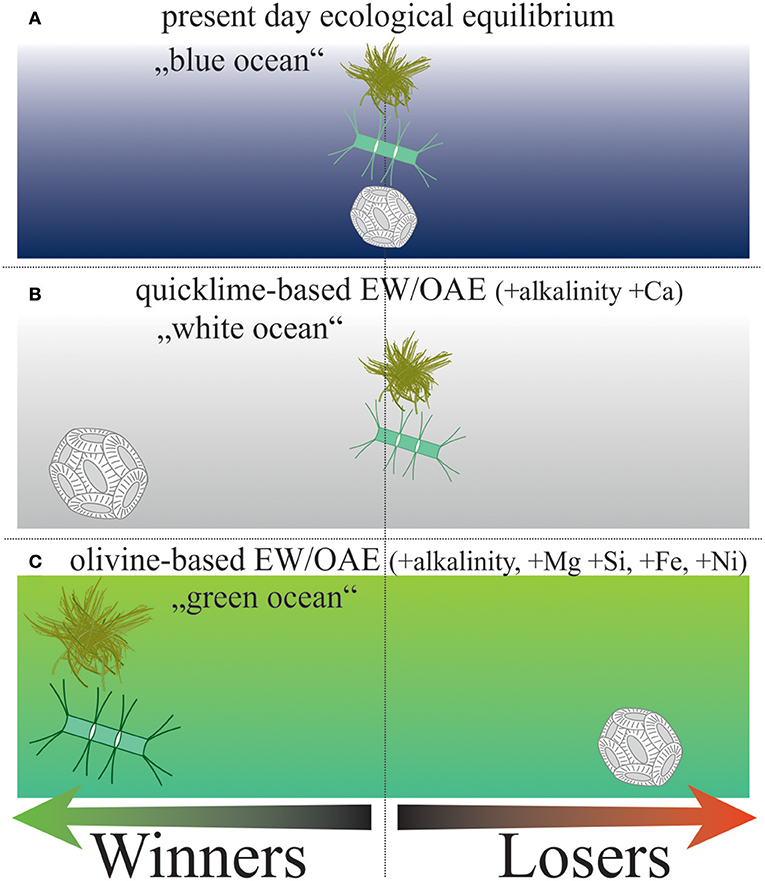
Figure 5. The “white or green ocean hypothesis.” This schematic drawing synthesizes the potential changes in the present day ecological equilibrium (A) under either quicklime-based (B) or olivine-based EW/OAE (C). = cyanobacteria;
= coccolithophores;
= diatoms.
When natural minerals such as olivine are used then the desired alkalinity increase coincides with a substantial DSi and trace metal enrichment (Box 2). In this scenario, global primary production would likely increase mostly due to Fe fertilization in Fe-limited open ocean regions (Köhler et al., 2013; Hauck et al., 2016). Silicifiers could profit from the additional DSi and strengthen their global dominance. Coccolithophores could be out-competed by silicifiers. N2-fixers could profit from Fe and Ni enrichment and reinforce primary production further until other nutrients (e.g., P) become limiting. This transformation under olivine-based EW/OAE could result in a more productive “green(er) ocean” (Figure 5C).
If ecological shifts toward a white(r) or green(er) ocean were shown to be realistic consequences of different EW/OAE schemes, they may influence: 1) if humankind is willing to accept changes of the affected ocean environments including their associated risks and co-benefits; 2) the choice of minerals to be used and amounts to be added in order to find the right balance between CDR efficacy and acceptability of EW/OAE.
Outlook
The risks and co-benefits discussed in this paper are based upon the presently available information on the most prominent dissolution products of the most widely considered source minerals (quicklime and silicate minerals such as olivine). Future research might reveal other minerals to be more suitable for EW/OAE and these minerals may be associated with other risks and co-benefits. Furthermore, the timing of mineral additions may become an important factor to consider in OAE schemes since side-effects may play out differently when additions occur in different seasons. Finally, we acknowledge that there are most certainly other risks/co-benefits that have been neglected in our discussion and the list will only become more complete once the hands-on research on this topic gained momentum.
Testing the “white or green ocean hypothesis” will require a broad and mechanistic understanding how dissolution products affect species and, more importantly, entire ecosystems. It needs the full scale of experimental approaches, including physiological studies, mesocosms, and modeling. Importantly, lessons learned from previous research efforts suggest that in situ surveys should be initiated early in a developing field to cover the ecological complexity of natural plankton communities (Riebesell and Gattuso, 2015). This could be realized by studying natural analogs such the Black Sea as an example for a high alkalinity marginal sea. Likewise, sites of intense natural weathering of basaltic rocks along volcanic islands may provide insights on more regional impacts (Hartmann et al., 2009). Studies of marine paleo-environments may also improve our understanding of EW/OAE, since there are episodes in the geological past where seawater chemistry had similarities to what could be expected under EW/OAE in the future [e.g., higher DNi (Glass and Dupont, 2017) or higher alkalinity (Tyrrell and Zeebe, 2004)].
Ultimately, it will be important to plan and eventually execute (sub-) mesoscale in situ OAE experiments since only experiments at the scale envisioned for OAE perturbations can provide a more comprehensive picture of ecosystem-wide impacts (Carpenter, 1996). Indeed, previous mesoscale iron enrichment studies have provided insights on the efficacy and potential side-effects of this NET that would probably not been revealed with conventional methodology (Boyd and Bressac, 2016). However, comprehensive risk assessments for environment including ethical considerations in an open public discourse would have to be made to assess whether such experimentation would be morally acceptable (Lawford-Smith and Currie, 2017; Oschlies and Klepper, 2017; Pidgeon and Spence, 2017; GESAMP, 2019).
Concerning legal aspects, there is currently some unclarity with respect to the governance for NETs (Boyd and Vivian, 2019). OAE may fall into either national regulation if activities were limited to the inland waters, or the global “London Convention (LC)/ London Protocol (LP),” treaties that regulate marine pollution from the dumping of wastes or other matter at sea [The International Maritime Organization (IMO) listed 87 members to the LC and 51 members to the LP where the latter was agreed to further modernize the LC and eventually replace it (July, 2019; www.imo.org)]. The LC/LP sets high hurdles on legitimate scientific research involving additions of minerals to the open oceans (e.g., ocean iron fertilization and potentially also open ocean alkalinity enhancement) but does not prohibit it (GESAMP, 2019). EW is not within the scope of the LC/LP because land-based sources of dissolution products would not be considered within their definition of “dumping” materials in the oceans. The assessment of risks and co-benefits of EW/OAE should therefore be paralleled by the development of a precise regulatory framework that is informed by the independent scientific community (Oschlies and Klepper, 2017; Gattuso et al., 2018; Boyd and Vivian, 2019).
Assessing EW/OAE impacts on ocean ecosystem is a new and understudied research branch in Biological Oceanography but the methodology and the scientific expertise is already largely established. For example, the widespread interest in the marine carbon cycle and ocean acidification has catalyzed the proliferation of global networks of stationary or robotic carbonate chemistry observation platforms which could be used to assess the success of OAE to store CO2 in seawater (e.g., http://www.goa-on.org or http://biogeochemical-argo.org). This constitutes an invaluable basis since only certified long-term storage (ideally >>1,000 years) can be used for carbon management. Furthermore, biology-centered ocean acidification research formed a well-trained research community that can easily design and perform experiments to investigate EW/OAE-related carbonate chemistry impacts on marine biota. Marine Si cycling and trace metal ecotoxicology are equally established research fields and the available knowledge of highly skilled scientists will become invaluable to determine risks and co-benefits of EW/OAE for the oceans. The key challenge is to bring together expertise from already established fields in Biological Oceanography in order to make the necessary progress within the limited timeframe given by the remaining carbon budget to stay below 2°C.
Data Availability Statement
Publicly available datasets were analyzed in this study. All references where the data is available is given in the corresponding text passages.
Author Contributions
LB conceptualized the research, compiled information in Table 1, composed the figures, and drafted the manuscript except for section risks and co-benefits of increased (Earth) alkaline metal concentrations. SG and RR drafted risks and co-benefits of increased (Earth) alkaline metal concentrations. All authors revised and improved the manuscript.
Conflict of Interest
The authors declare that the research was conducted in the absence of any commercial or financial relationships that could be construed as a potential conflict of interest.
Acknowledgments
We thank Jennifer Glass, Christopher Dupont, and the Royal Society of Chemistry for allowing us to use their Figure in our manuscript (Figure 4), the reviewers for their insightful comments, and Susan Hovorka for handling this manuscript. LB was thankful to Ulf Riebesell, Jan Taucher, Allanah Paul, David Keller, Andreas Oschlies, and Philip Boyd for stimulating discussions as well as for funding from GEOMAR Helmholtz Centre for Ocean Research Kiel and the Australian Research Council within a Laureate (FL160100131) granted to P. Boyd. RR, SG, and PR acknowledge the NERC consortium GGREW (NE/P01982X/1) for financial support. SG was supported by the Natural Environment Research Council (NERC) GW4+ Doctoral Training Partnership.
References
Amiotte Suchet, P., Probst, J.-L., and Ludwig, W. (2003). Worldwide distribution of continental rock lithology: implications for the atmospheric/soil CO2 uptake by continental weathering and alkalinity river transport to the oceans. Glob. Biogeochem. Cycles 17, 1038–1051. doi: 10.1029/2002GB001891
Anderson, K., and Peters, G. (2016). The trouble with negative emissions. Science 354, 182–183. doi: 10.1126/science.aah4567
Andersson, A. J., Mackenzie, F. T., and Bates, N. R. (2008). Life on the margin: implications of ocean acidification on Mg-calcite, high latitude and cold-water marine calcifiers. Mar. Ecol. Prog. Ser. 373, 265–273. doi: 10.3354/meps07639
Armbrust, E. V. (2009). The life of diatoms in the world's oceans. Nature 459, 185–192. doi: 10.1038/nature08057
Armstrong, R. A., Lee, C., Hedges, J. I., Honjo, S., and Wakeham, S. G. (2002). A new, mechanistic model for organic carbon fluxes in the ocean based on the quantitative association of POC with ballast minerals. Deep Sea Res. Part II 49, 219–236. doi: 10.1016/S0967-0645(01)00101-1
Bach, L. T. (2015). Reconsidering the role of carbonate ion concentration in calcification by marine organisms. Biogeosciences 12, 4939–4951. doi: 10.5194/bg-12-4939-2015
Bach, L. T., Boxhammer, T., Larsen, A., Hildebrandt, N., Schulz, K. G., and Riebesell, U. (2016). Influence of plankton community structure on the sinking velocity of marine aggregates. Glob. Biogeochem. Cycles 30, 1199–1214. doi: 10.1002/2016GB005372
Bach, L. T., Riebesell, U., Gutowska, M. A., Federwisch, L., and Schulz, K. G. (2015). A unifying concept of coccolithophore sensitivity to changing carbonate chemistry embedded in an ecological framework. Prog. Oceanogr. 135, 125–138. doi: 10.1016/j.pocean.2015.04.012
Bach, L. T., Riebesell, U., and Schulz, K. G. (2011). Distinguishing between the effects of ocean acidification and ocean carbonation in the coccolithophore Emiliania huxleyi. Limnol. Oceanogr. 56, 2040–2050. doi: 10.4319/lo.2011.56.6.2040
Beerling, D. J. (2017). Enhanced rock weathering: biological climate change mitigation with co-benefits for food security? Biol. Lett. 13, 4–7. doi: 10.1098/rsbl.2017.0149
Beerling, D. J., Leake, J. R., Long, S. P., Scholes, J. D., Ton, J., Nelson, P. N., et al. (2018). Farming with crops and rocks to address global climate, food and soil security. Nat. Plants 4, 138–147. doi: 10.1038/s41477-018-0108-y
Berner, R. A. (1975). The role of magnesium in the crystal growth of calcite and aragonite from sea water. Geochim. Cosmochim. Acta 39, 489–504. doi: 10.1016/0016-7037(75)90102-7
Boyd, P., and Newton, P. (1995). Evidence of the potential influence of planktonic community structure on the interannual variability of particulate organic carbon flux. Deep Sea Res. Part I 42, 619–639. doi: 10.1016/0967-0637(95)00017-Z
Boyd, P., and Vivian, C. (2019). Should we fertilize oceans or seed clouds? No one knows. Nature 570, 155–157. doi: 10.1038/d41586-019-01790-7
Boyd, P. W., and Bressac, M. (2016). Developing a test-bed for robust research governance of geoengineering: the contribution of ocean iron biogeochemistry. Philos. Trans. R. Soc. A Math. Phys. Eng. Sci. 374:20150299. doi: 10.1098/rsta.2015.0299
Boyd, P. W., and Ellwood, M. J. (2010). The biogeochemical cycle of iron in the ocean. Nat. Geosci. 3, 675–682. doi: 10.1038/ngeo964
Boyd, P. W., Jickells, T., Law, C. S., Blain, S., Boyle, E. A., Buesseler, K. O., et al. (2007). Mesoscale iron enrichment experiments 1993-2005: synthesis and future directions. Science 315, 612–617. doi: 10.1126/science.1131669
Braun, C., Merk, C., Pönitzsch, G., Rehdanz, K., and Schmidt, U. (2018). Public perception of climate engineering and carbon capture and storage in Germany: survey evidence. Clim. Policy 18, 471–484. doi: 10.1080/14693062.2017.1304888
Browning, T. J., Achterberg, E. P., Rapp, I., Engel, A., Bertrand, E. M., Tagliabue, A., et al. (2017). Nutrient co-limitation at the boundary of an oceanic gyre. Nature 551, 242–246. doi: 10.1038/nature24063
Brzezinski, M. A., Pride, C. J., Franck, V. M., Sigman, D. M., Sarmiento, J. L., Matsumoto, K., et al. (2002). A switch from Si(OH)4 to depletion in the glacial Southern Ocean. Geophys. Res. Lett. 29, 1–5. doi: 10.1029/2001GL014349
Burridge, A. K., Hörnlein, C., Janssen, A. W., Hughes, M., Bush, S. L., Marlétaz, F., et al. (2017). Time-calibrated molecular phylogeny of pteropods. PLoS ONE 12:e0177325. doi: 10.1371/journal.pone.0177325
Caldeira, K., and Rau, G. H. (2000). Accelerating carbonate dissolution to sequester carbon dioxide in the ocean: geochemical implications. Geophys. Res. Lett. 27, 225–228. doi: 10.1029/1999GL002364
Carafoli, E. (1987). Intracellular calcium homeostasis. Annu. Rev. Biochem. 56, 395–433. doi: 10.1146/annurev.bi.56.070187.002143
Carpenter, S. R. (1996). Microcosm experiments have limited relevance for community and ecosystem ecology. Ecology 77, 667–680. doi: 10.2307/2265490
Case, R. M., Eisner, D., Gurney, A., Jones, O., Muallem, S., and Verkhratsky, A. (2007). Evolution of calcium homeostasis: from birth of the first cell to an omnipresent signalling system. Cell Calcium 42, 345–350. doi: 10.1016/j.ceca.2007.05.001
Chrachri, A., Hopkinson, B. M., Flynn, K., Brownlee, C., and Wheeler, G. L. (2018). Dynamic changes in carbonate chemistry in the microenvironment around single marine phytoplankton cells. Nat. Commun. 9, 1–12. doi: 10.1038/s41467-017-02426-y
Ciais, P., Sabine, C., Bala, G., Bopp, L., Brovkin, V., Canadell, J., et al. (2013). “Carbon and other biogeochemical cycles,” in Climate Change 2013: The Physical Science Basis. Contribution of Working Group I to the Fifth Assessment Report of the Intergovernmental Panel on Climate Change, eds T. F. Stocker, D. Qin, G.-K. Plattner, M. Tignor, J. Allen, J. Boschung, et al. (Cambridge: Cambridge University Press, 465–570.
Conley, D. J., Frings, P. J., Fontorbe, G., Clymans, W., Stadmark, J., Hendry, K. R., et al. (2017). Biosilicification drives a decline of dissolved Si in the oceans through geologic time. Front. Mar. Sci. 4:397. doi: 10.3389/fmars.2017.00397
Cripps, G., Widdicombe, S., Spicer, J. I., and Findlay, H. S. (2013). Biological impacts of enhanced alkalinity in Carcinus maenas. Mar. Pollut. Bull. 71, 190–198. doi: 10.1016/j.marpolbul.2013.03.015
Cyronak, T., Schulz, K. G., and Jokiel, P. L. (2015). The Omega myth: what really drives lower calcification rates in an acidifying ocean. ICES J. Mar. Sci. 73, 558–562. doi: 10.1093/icesjms/fsv075
Davis, K. J., Dove, P. M., and De Yoreo, J. J. (2000). The role of Mg2+ as an impurity in calcite growth. Science 290, 1134–1137. doi: 10.1126/science.290.5494.1134
Dickson, A. G. (1981). An exact definition of total alkalinity and a procedure for the estimation of alkalinity and total inorganic carbon from titration data. Deep Res. 28A, 609–623. doi: 10.1016/0198-0149(81)90121-7
Dietzen, C., Harrison, R., and Michelsen-Correa, S. (2018). Effectiveness of enhanced mineral weathering as a carbon sequestration tool and alternative to agricultural lime: an incubation experiment. Int. J. Greenh. Gas Control 74, 251–258. doi: 10.1016/j.ijggc.2018.05.007
Doney, S. C., Fabry, V. J., Feely, R. A., and Kleypas, J. A. (2009). Ocean acidification: the other CO2 problem. Ann. Rev. Mar. Sci. 1, 169–192. doi: 10.1146/annurev.marine.010908.163834
Dugdale, R. C., Wilkerson, F. P., and Minas, H. J. (1995). The role of a silcate pump in driving new production. Deep Res. I 42, 697–719. doi: 10.1016/0967-0637(95)00015-X
Dupont, C. L., Buck, K. N., Palenik, B., and Barbeau, K. (2010). Nickel utilization in phytoplankton assemblages from contrasting oceanic regimes. Deep Res. Part I 57, 553–566. doi: 10.1016/j.dsr.2009.12.014
Dürr, H. H., Meybeck, M., Hartmann, J., Laruelle, G. G., and Roubeix, V. (2011). Global spatial distribution of natural riverine silica inputs to the coastal zone. Biogeosciences 8, 597–620. doi: 10.5194/bg-8-597-2011
Edwards, D. P., Lim, F., James, R. H., Pearce, C. R., Scholes, J., Freckleton, R. P., et al. (2017). Climate change mitigation: potential benefits and pitfalls of enhanced rock weathering in tropical agriculture. Biol. Lett. 13:20160715. doi: 10.1098/rsbl.2016.0715
Egge, J. K., and Jacobsen, A. (1997). Influence of silicate on particulate carbon production in phytoplankton. Mar. Ecol. Prog. Ser. 147, 219–230. doi: 10.3354/meps147219
Falkowski, P. G., Katz, M. E., Knoll, A. H., Quigg, A., Raven, J. A, Schofield, O., et al. (2004). The evolution of modern eukaryotic phytoplankton. Science 305, 354–360. doi: 10.1126/science.1095964
Fantle, M. S., and Tipper, E. T. (2014). Calcium isotopes in the global biogeochemical Ca cycle: implications for development of a Ca isotope proxy. Earth Sci. Rev. 129, 148–177. doi: 10.1016/j.earscirev.2013.10.004
Feng, E. Y., Koeve, W., Keller, D. P., and Oschlies, A. (2017). Model-based assessment of the CO2 sequestration potential of coastal ocean alkalinization. Earths Future 5, 1252–1266. doi: 10.1002/2017EF000659
Field, C. B., Behrenfeld, M. J., Randerson, J. T., and Falkowski, P. G. (1998). Primary production of the biosphere: integrating terrestrial and oceanic components. Science 281, 237–240. doi: 10.1126/science.281.5374.237
Flynn, K. J., Blackford, J. C., Baird, M. E., Raven, J. A., Clark, D. R., Beardall, J., et al. (2012). Changes in pH at the exterior surface of plankton with ocean acidification. Nat. Clim. Change 2, 510–513. doi: 10.1038/nclimate1489
Francois, R., Honjo, S., Krishfield, R., and Manganini, S. (2002). Factors controlling the flux of organic carbon to the bathypelagic zone of the ocean. Glob. Biogeochem. Cycles 16:1087. doi: 10.1029/2001GB001722
Friedrichs, L., Hörnig, M., Schulze, L., Bertram, A., Jansen, S., and Hamm, C. (2013). Size and biomechanic properties of diatom frustules influence food uptake by copepods. Mar. Ecol. Prog. Ser. 481, 41–51. doi: 10.3354/meps10227
Fujii, M., Yeung, A. C. Y., and Waite, T. D. (2015). Competitive effects of calcium and magnesium ions on the photochemical transformation and associated cellular uptake of iron by the freshwater cyanobacterial phytoplankton Microcystis aeruginosa. Environ. Sci. Technol. 49, 9133–9142. doi: 10.1021/acs.est.5b01583
Fuss, S., Jones, C. D., Kraxner, F., Peters, G. P., Smith, P., Tavoni, M., et al. (2016). Research priorities for negative emissions. Environ. Res. Lett. 11:115007. doi: 10.1088/1748-9326/11/11/115007
Fuss, S., Lamb, W. F., Callaghan, M. W., Hilaire, J., Creutzig, F., Amann, T., et al. (2018). Negative emissions — Part 2 : costs, potentials and side effects. Environ. Res. Lett. 12:063002. doi: 10.1088/1748-9326/aabf9f
Gabe, U., and Rodella, A. A. (1999). Trace elements in Brazilian agricultural limestones and mineral fertilizers. Commun. Soil Sci. Plant Anal. 30, 605–620. doi: 10.1080/00103629909370231
Gaillardet, J., Viers, J., and Dupré, B. (2014). “Trace elements in river waters,” in Treatise on Geochemistry: Surface and Groundwater, Weathering and Soils, ed J. I. Drever (Amsterdam: Elsevier), 195–236. doi: 10.1016/B978-0-08-095975-7.00507-6
Gall, L., Williams, H. M., Siebert, C., Halliday, A. N., Herrington, R. J., and Hein, J. R. (2013). Nickel isotopic compositions of ferromanganese crusts and the constancy of deep ocean inputs and continental weathering effects over the Cenozoic. Earth Planet. Sci. Lett. 375, 148–155. doi: 10.1016/j.epsl.2013.05.019
Galván-Ruiz, M., Hernández, J., Baños, L., Noriega-Montes, J., and Rodríguez-García, M. E. (2009). Characterization of calcium carbonate, calcium oxide, and calcium hydroxide as starting point to the improvement of lime for their use in construction. J. Mater. Civ. Eng. 21, 694–698. doi: 10.1061/(ASCE)0899-1561(2009)21:11(694)
Gattuso, J.-P., Magnan, A. K., Bopp, L., Cheung, W. W. L., Duarte, C. M., Hinkel, J., et al. (2018). Ocean solutions to address climate change and its effects on marine ecosystems. Front. Mar. Sci. 5:337. doi: 10.3389/fmars.2018.00337
Gattuso, J. P., Magnan, A., Billé, R., Cheung, W. W. L., Howes, E. L., Joos, F., et al. (2015). Contrasting futures for ocean and society from different anthropogenic CO2 emissions scenarios. Science 349:aac4722. doi: 10.1126/science.aac4722
GESAMP (2019). “High level review of a wide range of proposed marine geoengineering techniques GESAMP working group 41. No 98,” in IMO/FAO/UNESCO-IOC/UNIDO/WMO/IAEA/UN/UN Environment/ UNDP/ISA Joint Group of Experts on the Scientific Aspects of Marine Environmental Protection, eds P. W. Boyd and C. Vivian (London: International Maritime Organization), 144.
Giordano, M., Beardall, J., and Raven, J. A. (2005). CO2 concentrating mechanisms in algae: mechanisms, environmental modulation, and evolution. Annu. Rev. Plant Biol. 56, 99–131. doi: 10.1146/annurev.arplant.56.032604.144052
Glass, J. B., and Dupont, C. L. (2017). “Oceanic nickel biogeochemistry and the evolution of nickel use,” in The Biological Chemistry of Nickel, eds D. Zamble, M. Rowinska-Zyrek, and H. Kozlowski (Croydon: The Royal Society of Chemistry, 12–26.
Goldman, J. (1999). Inorganic carbon availability and the growth of large marine diatoms. Mar. Ecol. Prog. Ser. 180, 81–91. doi: 10.3354/meps180081
González, M. F., and Ilyina, T. (2016). Impacts of artificial ocean alkalinization on the carbon cycle and climate in earth system simulations. Geophys. Res. Lett. 43, 6493–6502. doi: 10.1002/2016GL068576
Gore, S., Renforth, P., and Perkins, R. (2018). The potential environmental response to increasing ocean alkalinity for negative emissions. Mitig. Adapt. Strat. Glob. Change doi: 10.1007/s11027-018-9830-z. [Epub ahead of print].
Goyet, C., Bradshaw, A. L., and Brewer, P. G. (1991). The carbonate system in the Black Sea. Deep Sea Res. Part A 38, S1049–S1068. doi: 10.1016/S0198-0149(10)80023-8
Griffioen, J. (2017). Enhanced weathering of olivine in seawate : the efficiency as revealed by thermodynamic scenario analysis. Sci. Total Environ. 575, 536–544. doi: 10.1016/j.scitotenv.2016.09.008
Guiry, M. D., Guiry, G. M., Morrison, L., Rindi, F., Miranda, S. V., Mathieson, A. C., et al. (2014). AlgaeBase: an on-line resource for algae. BioOne 35, 105–115. doi: 10.7872/crya.v35.iss2.2014.105
Guo, J., Wang, F., Vogt, R. D., Zhang, Y., and Liu, C. Q. (2015). Anthropogenically enhanced chemical weathering and carbon evasion in the Yangtze Basin. Sci. Rep. 5, 1–8. doi: 10.1038/srep11941
Gutjahr, A., Dabringhaus, H., and Lacmann, R. (1996). Studies of the growth and dissolution kinetics of the CaCo3 polymorphs calcite and aragonite II. The influence of divalent cation additives on the growth and dissolution rates. J. Cryst. Growth 158, 310–315. doi: 10.1016/0022-0248(95)00447-5
Hamm, C. E., Merkel, R., Springer, O., Jurkojc, P., Maiert, C., Prechtelt, K., et al. (2003). Architecture and material properties of diatom shells provide effective mechanical protection. Nature 421, 841–843. doi: 10.1038/nature01416
Hangx, S. J. T., and Spiers, C. J. (2009). Coastal spreading of olivine to control atmospheric CO2 concentrations: a critical analysis of viability. Int. J. Greenh. Gas Control 3, 757–767. doi: 10.1016/j.ijggc.2009.07.001
Hansen, P. J. (2002). Effect of high pH on the growth and survival of marine phytoplankton: implications for species succession. Aquat. Microb. Ecol. 28, 279–288. doi: 10.3354/ame028279
Hartmann, J., Jansen, N., Dürr, H. H., Kempe, S., and Köhler, P. (2009). Global CO2-consumption by chemical weathering: what is the contribution of highly active weathering regions? Glob. Planet. Change 69, 185–194. doi: 10.1016/j.gloplacha.2009.07.007
Hartmann, J., West, A. J., Renforth, P., Köhler, P., De La Rocha, C. L., Wolf-gladrow, D., et al. (2013). Enhanced chemical weathering as a geoengineering strategy to reduce atmospheric carbon dioxide, supply nutirents, and mitigate ocean acidification. Rev. Geophys. 51, 113–149. doi: 10.1002/rog.20004
Harvey, L. D. D. (2008). Mitigating the atmospheric CO2 increase and ocean acidification by adding limestone powder to upwelling regions. J. Geophys. Res. Oceans 113, 1–21. doi: 10.1029/2007JC004373
Hauck, J., Köhler, P., Wolf-Gladrow, D., and Völker, C. (2016). Iron fertilisation and century-scale effects of open ocean dissolution of olivine in a simulated CO2 removal experiment. Environ. Res. Lett. 11:24007. doi: 10.1088/1748-9326/11/2/024007
Hay, B. J. (1988). Sediment accumulation in the central western part of the Black Sea over the last 5100 years. Paleoocenography 3, 491–508. doi: 10.1029/PA003i004p00491
Heck, V., Gerten, D., Lucht, W., and Popp, A. (2018). Biomass-based negative emissions difficult to reconcile with planetary boundaries. Nat. Clim. Change 8, 151–155. doi: 10.1038/s41558-017-0064-y
Ho, T. Y. (2013). Nickel limitation of nitrogen fixation in Trichodesmium. Limnol. Oceanogr. 58, 112–120. doi: 10.4319/lo.2013.58.1.0112
Holzer, M., Primeau, W., Devries, T., and Matear, R. (2014). The Southern Ocean silicon trap: data-constrained estimates of regenerated silicic acid, trapping efficiencies, and global transport paths. J. Geophys. Res. Oceans 119, 313–331. doi: 10.1002/2013JC009356
Honjo, S., Manganini, S. J., Krishfield, R. A, and Francois, R. (2008). Particulate organic carbon fluxes to the ocean interior and factors controlling the biological pump: a synthesis of global sediment trap programs since 1983. Prog. Oceanogr. 76, 217–285. doi: 10.1016/j.pocean.2007.11.003
Howes, E. L., Bednaršek, N., Buedenbender, J., Comeau, S., Doubleday, A., Gallager, S. M., et al. (2014). Sink and swim: a status review of thecosome pteropod culture techniques. J. Plankton Res. 36, 299–315. doi: 10.1093/plankt/fbu002
Humborg, C., Ittekkot, V., Cociasu, A., and von Bodungen, B. (1997). Effect of Danube River dam on Black Sea biogeochemistry and ecosystem structure. Nature 386, 385–388. doi: 10.1038/386385a0
Ilyina, T., Wolf-Gladrow, D., Munhoven, G., and Heinze, C. (2013). Assessing the potential of calcium-based artificial ocean alkalinization to mitigate rising atmospheric CO2 and ocean acidification. Geophys. Res. Lett. 40, 5909–5914. doi: 10.1002/2013GL057981
Järup, L. (2003). Hazards of heavy metal contamination. Br. Med. Bull. 68, 167–182. doi: 10.1093/bmb/ldg032
Jickells, T. D., An, Z. S., Andersen, K. K., Baker, A. R., Bergametti, G., Brooks, N., et al. (2005). Global iron connections between desert dust, ocean biogeochemistry, and climate. Science 308, 67–71. doi: 10.1126/science.1105959
Jokiel, P. L. (2011). The reef coral two compartment proton flux model: a new approach relating tissue-level physiological processes to gross corallum morphology. J. Exp. Mar. Bio. Ecol. 409, 1–12. doi: 10.1016/j.jembe.2011.10.008
Kapsenberg, L., Alliouane, S., Gazeau, F., Mousseau, L., and Gattuso, J. P. (2017). Coastal ocean acidification and increasing total alkalinity in the northwestern Mediterranean Sea. Ocean Sci. 13, 411–426. doi: 10.5194/os-13-411-2017
Kaushal, S. S., Likens, G. E., Pace, M. L., Utz, R. M., Haq, S., Gorman, J., et al. (2018). Freshwater salinization syndrome on a continental scale. Proc. Natl. Acad. Sci. 115:201711234. doi: 10.1073/pnas.1711234115
Keller, D. P., Feng, E. Y., and Oschlies, A. (2014). Potential climate engineering effectiveness and side effects during a high carbon dioxide-emission scenario. Nat. Commun. 5, 1–11. doi: 10.1038/ncomms4304
Key, R. M., Kozyr, A., Sabine, C. L., Lee, K., Wanninkhof, R., Bullister, J. L., et al. (2004). A global ocean carbon climatology: results from Global Data Analysis Project (GLODAP). Glob. Biogeochem. Cycles 18:GB4031. doi: 10.1029/2004GB002247
Kheshgi, H. S. (1995). Sequestering atmospheric carbon dioxide by increasing ocean alkalinity. Energy 20, 915–922. doi: 10.1016/0360-5442(95)00035-F
Klaas, C., and Archer, D. E. (2002). Association of sinking organic matter with various types of mineral ballast in the deep sea : implications for the rain ratio. Glob. Biogeochem. Cycles 16, 1–14. doi: 10.1029/2001GB001765
Köhler, P., Abrams, J. F., Völker, C., Hauck, J., and Wolf-Gladrow, D. A. (2013). Geoengineering impact of open ocean dissolution of olivine on atmospheric CO2, surface ocean pH and marine biology. Environ. Res. Lett. 8:014009. doi: 10.1088/1748-9326/8/1/014009
Köhler, P., Hartmann, J., and Wolf-Gladrow, D. A. (2010). Geoengineering potential of artificially enhanced silicate weathering of olivine. Proc. Natl. Acad. Sci. U.S.A. 107, 20228–20233. doi: 10.1073/pnas.1000545107
Konhauser, K. O., Pecoits, E., Lalonde, S. V., Papineau, D., Nisbet, E. G., Barley, M. E., et al. (2009). Oceanic nickel depletion and a methanogen famine before the Great Oxidation Event. Nature 458, 750–753. doi: 10.1038/nature07858
Kopelevich, O., Burenkov, V., Sheberstov, S., Vazyulya, S., Kravchishina, M., Pautova, L., et al. (2014). Satellite monitoring of coccolithophore blooms in the Black Sea from ocean color data. Remote Sens. Environ. 146, 113–123. doi: 10.1016/j.rse.2013.09.009
Krachler, R., Krachler, R. F., Wallner, G., Hann, S., Laux, M., Cervantes Recalde, M. F., et al. (2015). River-derived humic substances as iron chelators in seawater. Mar. Chem. 174, 85–93. doi: 10.1016/j.marchem.2015.05.009
Kroeker, K. J., Kordas, R. L., Crim, R. N., and Singh, G. G. (2010). Meta-analysis reveals negative yet variable effects of ocean acidification on marine organisms. Ecol. Lett. 13, 1419–1434. doi: 10.1111/j.1461-0248.2010.01518.x
Kwon, E. Y., Primeau, F., and Sarmiento, J. L. (2009). The impact of remineralization depth on the air–sea carbon balance. Nat. Geosci. 2, 630–635. doi: 10.1038/ngeo612
Laruelle, G. G., Roubeix, V., Sferratore, A., Brodherr, B., Ciuffa, D., Conley, D. J., et al. (2009). Anthropogenic perturbations of the silicon cycle at the global scale: key role of the land-ocean transition. Glob. Biogeochem. Cycles 23, 1–17. doi: 10.1029/2008GB003267
Lawford-Smith, H., and Currie, A. (2017). Accelerating the carbon cycle: the ethics of enhanced weathering. Biol. Lett. 13:20160859. doi: 10.1098/rsbl.2016.0859
Le Moigne, F. A. C., Pabortsava, K., Marcinko, C. L. J., Martin, P., and Sanders, R. J. (2014). Where is mineral ballast important for surface export of particulate organic carbon in the ocean? Geophys. Res. Lett. 41, 1–9. doi: 10.1002/2014GL061678
Le Quéré, C., Andrew, R. M., Canadell, J. G., Sitch, S., Korsbakken, J. I., Peters, G. P., et al. (2016). Global carbon budget 2016. Earth Syst. Sci. Data 8, 605–649. doi: 10.5194/essd-8-605-2016
Lebrato, M., Iglesias-Rodriguez, D., Feely, R., Greeley, D., Jones, D., Suarez-Bosche, N., et al. (2010). Global contribution of echinoderms to the marine carbon cycle: a re-assessment of the oceanic CaCO3 budget and the benthic compartments. Ecol. Monogr. 80, 441–467. doi: 10.1890/09-0553.1
Lenton, A., Matear, R. J., Keller, D. P., Scott, V., and Vaughan, N. E. (2018). Assessing carbon dioxide removal through global and regional ocean alkalinization under high and low emission pathways. Earth Syst. Dyn. 9, 339–357. doi: 10.5194/esd-9-339-2018
Lin, A. (2013). Does geoengineering present a moral hazard? Ecol. Law Q. 40, 673–712. doi: 10.15779/Z38JP1J
Liu, H., Chen, M., Zhu, F., and Harrison, P. J. (2016). Effect of diatom silica content on copepod grazing, growth and reproduction. Front. Mar. Sci. 3:89. doi: 10.3389/fmars.2016.00089
Lueker, T. J., Dickson, A. G., and Keeling, C. D. (2000). Ocean pCO2 calculated from dissolved inorganic carbon, alkalinity, and equations for K1 and K2: validation based on laboratory measurements of CO2 in gas and seawater at equilibrium. Mar. Chem. 70, 105–119. doi: 10.1016/S0304-4203(00)00022-0
Martin-Jézéquel, V., Hildebrand, M., and Brzezinski, M. A. (2000). Review silicon metabolism in diatoms: implications for growth. J. Phycol. 36, 821–840. doi: 10.1046/j.1529-8817.2000.00019.x
Matsumoto, K., Sarmiento, J. L., and Brzezinski, M. A. (2002). Silicic acid leakage from the Southern Ocean : a possible explanation for glacial atmospheric pCO2. Glob. Biogeochem. Cycles 16, 5-1–5-23. doi: 10.1029/2001GB001442
Mayes, W. M., Younger, P. L., and Aumônier, J. (2008). Hydrogeochemistry of alkaline steel slag leachates in the UK. Water Air Soil Pollut. 195, 35–50. doi: 10.1007/s11270-008-9725-9
Meier, A., Bonaldi, E., Cella, G. M., Lipinski, W., and Wuillemin, D. (2006). Solar chemical reactor technology for industrial production of lime. Sol. Energy 80, 1355–1362. doi: 10.1016/j.solener.2005.05.017
Meysman, F. J. R., and Montserrat, F. (2017). Negative CO2 emissions via enhanced silicate weathering in coastal environments. Biol. Lett. 13:20160905. doi: 10.1098/rsbl.2016.0905
Milliman, J. D., Troy, P. J., Balch, W. M., Adams, A. K., Li, Y.-H., and Mackenzie, F. T. (1999). Biologically mediated dissolution of calcium carbonate above the chemical lysocline? Deep Sea Res. Part I 46, 1653–1669. doi: 10.1016/S0967-0637(99)00034-5
Mills, M. M., Ridame, C., Davey, M., La Roche, J., and Geider, R. J. (2004). Iron and phosphorus co-limit nitrogen fixation in the eastern tropical North Atlantic. Nature 429, 292–294. doi: 10.1038/nature02550
Minx, J. C., Lamb, W. F., Callaghan, M. W., Fuss, S., Hilaire, J., Creutzig, F., et al. (2018). Negative emissions — Part 1 : research landscape and synthesis. Environ. Res. Lett. 13:053001. doi: 10.1088/1748-9326/aabf9b
Monteiro, F. M., Bach, L. T., Brownlee, C., Bown, P., Rickaby, R. E. M., Poulton, A. J., et al. (2016). Why marine phytoplankton calcify (supplement). Sci. Adv. 2:e1501822. doi: 10.1126/sciadv.1501822
Montserrat, F., Renforth, P., Hartmann, J., Leermakers, M., Knops, P., and Meysman, F. J. R. (2017). Olivine dissolution in seawater: implications for CO2 sequestration through enhanced weathering in coastal environments. Environ. Sci. Technol. 51, 3960–3972. doi: 10.1021/acs.est.6b05942
Moore, C. M., Mills, M. M., Arrigo, K. R., Berman-Frank, I., Bopp, L., Boyd, P. W., et al. (2013). Processes and patterns of oceanic nutrient limitation. Nat. Geosci. 6, 701–710. doi: 10.1038/ngeo1765
Moosdorf, N., Renforth, P., and Hartmann, J. (2014). Carbon dioxide efficiency of terrestrial enhanced weathering. Environ. Sci. Technol. 48, 4809–4816. doi: 10.1021/es4052022
Müller, J. D., Schneider, B., and Rehder, G. (2016). Long-term alkalinity trends in the Baltic Sea and their implications for CO2-induced acidification. Limnol. Oceanogr. 61, 1984–2002. doi: 10.1002/lno.10349
Myhre, G., Shindell, D., Bréon, F., Collins, W., Fuglestvedt, J., Huang, J., et al. (2013). “Anthropogenic and natural radiative forcing,” in Climate Change 2013: The Physical Science Basis. Contribution of Working Group I to the Fifth Assessment Report of the Intergovernmental Panel of Climate Change, eds T. F. Stocker, D. Qin, G.-K. Plattner, M. Tignor, J. Allen, J. Boschung, et al. (Cambridge; New York, NY: Cambridge University Press, 658–740.
Nelson, D. M., Tréguer, P., Brzezinski, M. A., Leynaert, A., and Quéguiner, B. (1995). Production and dissolution of biogenic silica in the ocean: revised global estimates, comparison with regional data and relationship to biogenic sedimentation. Glob. Biogeochem. Cycles 9, 359–372. doi: 10.1029/95GB01070
Nemet, G. F., Callaghan, M. W., Creutzig, F., Fuss, S., Hartmann, J., Hilaire, J., et al. (2018). Negative emissions — Part 3: Innovation and upscaling. Environ. Res. Lett. 13:06300. doi: 10.1088/1748-9326/aabff4
Nieminen, T. M., Ukonmaanaho, L., Rausch, N., and Shotyk, W. (2007). “Biogeochemistry of nickel and its release into the envrionment,” in Nickeln and Its Surprising Impact in Nature, eds. A. Sigel, H. Sigel, and R. K. O. Sigel (Chichester: Wiley-VCH, 1–30.
Oelkers, E. H., Declercq, J., Saldi, G. D., Gislason, S. R., and Schott, J. (2018). Olivine dissolution rates: a critical review. Chem. Geol. 500, 1–19. doi: 10.1016/j.chemgeo.2018.10.008
Orr, J. C., Fabry, V. J., Aumont, O., Bopp, L., Doney, S. C., Feely, R. A., et al. (2005). Anthropogenic ocean acidification over the twenty-first century and its impact on calcifying organisms. Nature 437, 681–686. doi: 10.1038/nature04095
Oschlies, A., and Klepper, G. (2017). Research for assessment, not deployment, of climate engineering: the German Research Foundation's Priority Program SPP 1689. Earth's Fut. 5, 128–134. doi: 10.1002/2016EF000446
Oschlies, A., Koeve, W., Rickels, W., and Rehdanz, K. (2010). Side effects and accounting aspects of hypothetical large-scale Southern Ocean iron fertilization. Biogeosciences 7, 4014–4035. doi: 10.5194/bg-7-4017-2010
Pacala, S., Al-Kaisi, M., Barteau, M., Belmont, E., Benson, S., Birdsey, R., et al. (2019). National Academies of Sciences, Engineering, and Medicine. Negative Emissions Technologies and Reliable Sequestration: A Research Agenda. Washington, DC: The National Academies Press.
Paquay, F. S., and Zeebe, R. E. (2013). Assessing possible consequences of ocean liming on ocean pH, atmospheric CO2 concentration and associated costs. Int. J. Greenh. Gas Control 17, 183–188. doi: 10.1016/j.ijggc.2013.05.005
Pedersen, M. F., and Hansen, P. J. (2003). Effects of high pH on the growth and survival of six marine heterotrophic protists. Mar. Ecol. Prog. Ser. 260, 33–41. doi: 10.3354/meps260033
Peters, G. P. (2016). The “best available science” to inform 1.5°C policy choices. Nat. Clim. Change 6, 646–649. doi: 10.1038/nclimate3000
Pidgeon, N. F., and Spence, E. (2017). Perceptions of enhanced weathering as a biological negative emissions option. Biol. Lett. 13:20170024. doi: 10.1098/rsbl.2017.0024
Pokrovsky, O. S., and Schott, J. (2000). Forsterite surface composition in aqueous solutions: a combined potentiometric, elektrokinetic, and spectroscopic approach. Geochim. Cosmochim. Acta 64, 3299–3312. doi: 10.1016/S0016-7037(00)00435-X
Primeau, F. W., Holzer, M., and DeVries, T. (2013). Southern Ocean nutrient trapping and the efficiency of the biological pump. J. Geophys. Res. Ocean. 118, 2547–2564. doi: 10.1002/jgrc.20181
Ragueneau, O., Tréguer, P., Leynaert, A., Anderson, R. F., Brzezinski, M. A., DeMaster, D. J., et al. (2000). A review of the Si cycle in the modern ocean: recent progress and missing gaps in the application of biogenic opal as a paleoproductivity proxy. Glob. Planet. Change 26, 317–365. doi: 10.1016/S0921-8181(00)00052-7
Rau, G. H., Carroll, S. A., Bourcier, W. L., Singleton, M. J., Smith, M. M., and Aines, R. D. (2013). Direct electrolytic dissolution of silicate minerals for air CO2 mitigation and carbon-negative H2 production. Proc. Natl. Acad. Sci. U.S.A. 110, 10095–10100. doi: 10.1073/pnas.1222358110
Rau, G. H., McLeod, E. L., and Hoegh-Guldberg, O. (2012). The need for new ocean conservation strategies in a high-carbon dioxide world. Nat. Clim. Change 2, 720–724. doi: 10.1038/nclimate1555
Raymond, P. A., Hartmann, J., Lauerwald, R., Sobek, S., McDonald, C., Hoover, M., et al. (2013). Global carbon dioxide emissions from inland waters. Nature 503, 355–359. doi: 10.1038/nature12760
Renforth, P. (2019). The negative emission potential of alkaline materials. Nat. Commun. 10:1401. doi: 10.1038/s41467-019-09475-5
Renforth, P., and Henderson, G. (2017). Assessing ocean alkalinity for carbon sequestration. Rev. Geophys. 55, 636–674. doi: 10.1002/2016RG000533
Renforth, P., Jenkins, B. G., and Kruger, T. (2013). Engineering challenges of ocean liming. Energy 60, 442–452. doi: 10.1016/j.energy.2013.08.006
Renforth, P., and Kruger, T. (2013). Coupling mineral carbonation and ocean liming. Energy Fuels 27, 4199–4207. doi: 10.1021/ef302030w
Riebesell, U., Bach, L. T., Bellerby, R. G. J., Monsalve, J. R. B., Boxhammer, T., Czerny, J., et al. (2017). Competitive fitness of a predominant pelagic calcifier impaired by ocean acidification. Nat. Geosci. 10, 19–23. doi: 10.1038/ngeo2854
Riebesell, U., and Gattuso, J.-P. (2015). Lessons learned from ocean acidification research. Nat. Clim. Change 5, 12–14. doi: 10.1038/nclimate2456
Riebesell, U., Körtzinger, A., and Oschlies, A. (2009). Sensitivities of marine carbon fluxes to ocean change. Proc. Natl. Acad. Sci. U.S.A. 106, 20602–20609. doi: 10.1073/pnas.0813291106
Riebesell, U., Wolf-Gladrow, D. A., and Smetacek, V. (1993). Carbon dioxide limitation of marine phytoplankton growth rates. Nature 361, 249–251. doi: 10.1038/361249a0
Ries, J. B. (2010). Review: geological and experimental evidence for secular variation in seawater Mg/Ca (calcite-aragonite seas) and its effects on marine biological calcification. Biogeosciences 7, 2795–2849. doi: 10.5194/bg-7-2795-2010
Rockström, J., Gaffney, O., Rogelj, J., Meinshausen, M., Nakicenovic, N., and Schellnhuber, H. J. (2017). A roadmap for rapid decarbonization. Science 355, 1269–1271. doi: 10.1126/science.aah3443
Rogan, N., Achterberg, E., Le Moigne, F., Marsay, C., and Tagliabue, A. (2016). Volcanic ash as an ocean iron source and sink. Geophys. Res. Lett. 53, 2732–2740. doi: 10.1002/2016GL067905
Rogelj, J., Schaeffer, M., Friedlingstein, P., Gillett, N. P., Van Vuuren, D. P., Riahi, K., et al. (2016). Differences between carbon budget estimates unravelled. Nat. Clim. Change 6, 245–252. doi: 10.1038/nclimate2868
Romani, A. M. P. (2011). Intracellular magnesium homeostasis. Arch. Biochem. Biophys. 512, 1–23. doi: 10.1016/j.abb.2011.05.010
Rost, B., Riebesell, U., and Sültemeyer, D. (2006). Carbon acquisition of marine phytoplankton: effect of photoperiod length. Limnol. Oceanogr. 51, 12–20. doi: 10.4319/lo.2006.51.1.0012
Sanderson, B. M., O'Neill, B. C., and Tebaldi, C. (2016). What would it take to achieve the Paris temperature targets? Geophys. Res. Lett. 43, 7133–7142. doi: 10.1002/2016GL069563
Sarmiento, J. L., and Gruber, N. (2006). Ocean Biogeochemical Dynamics. Princeton: Princeton University Press.
Sarmiento, J. L., Gruber, N., Brzezinski, M. A., and Dunne, J. P. (2004). High-latitude controls of thermocline nutrients and low latitude biological productivity. Nature 427, 56–60. doi: 10.1038/nature02127
Sarthou, G., Timmermans, K. R., Blain, S., and Tréguer, P. (2005). Growth physiology and fate of diatoms in the ocean: a review. J. Sea Res. 53, 25–42. doi: 10.1016/j.seares.2004.01.007
Schellnhuber, H. J., Rahmstorf, S., and Winkelmann, R. (2016). Why the right climate target was agreed in Paris. Nat. Clim. Change 6, 649–653. doi: 10.1038/nclimate3013
Schlitzer, R., Anderson, R. F., Dodas, E. M., Lohan, M., Geibert, W., Tagliabue, A., et al. (2018). The GEOTRACES intermediate data product 2017. Chem. Geol. 493, 210–223. doi: 10.1016/j.chemgeo.2018.05.040
Schuiling, R. D., and Krijgsman, P. (2006). Enhanced weathering: an effective and cheap tool to sequester CO2. Clim. Change 74, 349–354. doi: 10.1007/s10584-005-3485-y
Segev, E., and Erez, J. (2006). Effect of Mg/Ca ratio in seawater on shell composition in shallow benthic foraminifera. Geochem. Geophys. Geosyst. 7, 1–8. doi: 10.1029/2005GC000969
Sett, S., Bach, L. T., Schulz, K. G., Koch-Klavsen, S., Lebrato, M., and Riebesell, U. (2014). Temperature modulates coccolithophorid sensitivity of growth, photosynthesis and calcification to increasing seawater pCO2. PLoS ONE 9:e88308. doi: 10.1371/journal.pone.0088308
Shue, H. (2017). Climate dreaming: negative emissions, risk transfer, and irreversibility. J. Hum. Rights Environ. 1–22. doi: 10.2139/ssrn.2940987
Šiler, P., Kolárová, I., Bednárek, J., Janča, M., Musil, P., and Opravil, T. (2018). “The possibilities of analysis of limestone chemical composition,” in IOP Conference Series: Materials Science and Engineering. 379, 1–6.
Simkin, T., and Smith, J. V. (1970). Minor-element distribution in Olivine. J. Geol. 78, 304–325. doi: 10.1086/627519
Sloyan, B. M., and Rintoul, S. R. (2001). Circulation, renewal, and modification of Antarctic mode and intermediate water. J. Phys. Oceanogr. 31, 1005–1030. doi: 10.1175/1520-0485(2001)031<1005:CRAMOA>2.0.CO;2
Smith, P., Davis, S. J., Creutzig, F., Fuss, S., Minx, J., Gabrielle, B., et al. (2016). Biophysical and economic limits to negative CO2 emissions. Nat. Clim. Change 6, 42–50. doi: 10.1038/nclimate2870
Sommer, U., Adrian, R., De Senerpont Domis, L., Elser, J. J., Gaedke, U., Ibelings, B., et al. (2012). Beyond the Plankton Ecology Group (PEG) Model: mechanisms driving plankton succession. Annu. Rev. Ecol. Evol. Syst. 43, 429–448. doi: 10.1146/annurev-ecolsys-110411-160251
Stanley, S. M., and Hardie, L. A. (1998). Secular oscillations in the carbonate mineralogy of reef-building and sediment-producing organisms driven by tectonically forced shifts in seawater chemistry. Palaeogeogr. Palaeoclimatol. Palaeoecol. 144, 3–19. doi: 10.1016/S0031-0182(98)00109-6
Stanley, S. M., Ries, J. B., and Hardie, L. A. (2005). Seawater chemistry, coccolithophore population growth, and the origin of Cretaceous chalk. Geology 33, 593–596. doi: 10.1130/G21405.1
Strauss, S. Y. (1991). Effects in community ecology: their definition, study and importance. Trends Ecol. Evol. 6, 206–210. doi: 10.1016/0169-5347(91)90023-Q
Strefler, J., Amann, T., Bauer, N., Kriegler, E., and Hartmann, J. (2018). Potential and costs of carbon dioxide removal by enhanced weathering of rocks. Environ. Res. Lett. 13:034010. doi: 10.1088/1748-9326/aaa9c4
Tagliabue, A., Bowie, A. R., Boyd, P. W., Buck, K. N., Johnson, K. S., and Saito, M. A. (2017). The integral role of iron in ocean biogeochemistry. Nature 543, 51–59. doi: 10.1038/nature21058
Takahashi, T., Sutherland, S. C., Chipman, D. W., Goddard, J. G., Ho, C., Newberger, T., et al. (2014). Climatological distributions of pH, pCO2, total CO2, alkalinity, and CaCO3 saturation in the global surface ocean, and temporal changes at selected locations. Mar. Chem. 164, 95–125. doi: 10.1016/j.marchem.2014.06.004
Taylor, L. L., Quirk, J., Thorley, R. M. S., Kharecha, P. A., Hansen, J., Ridgwell, A., et al. (2016). Enhanced weathering strategies for stabilizing climate and averting ocean acidification. Nat. Clim. Change 6, 402–406. doi: 10.1038/nclimate2882
Tipper, E. T., Galy, A., Gaillardet, J., Bickle, M. J., Elderfield, H., and Carder, E. A. (2006). The magnesium isotope budget of the modern ocean: constraints from riverine magnesium isotope ratios. Earth Planet. Sci. Lett. 250, 241–253. doi: 10.1016/j.epsl.2006.07.037
Tréguer, P., Bowler, C., Moriceau, B., Dutkiewicz, S., Gehlen, M., Aumont, O., et al. (2018). Influence of diatom diversity on the ocean biological carbon pump. Nat. Geosci. 11, 27–37. doi: 10.1038/s41561-017-0028-x
Tréguer, P. J., and De La Rocha, C. L. (2013). The world ocean silica cycle. Ann. Rev. Mar. Sci. 5, 477–501. doi: 10.1146/annurev-marine-121211-172346
Tyrrell, T., and Merico, A. (2004). “Emiliania huxleyi: bloom observations and the conditions that induce them,” in Coccolithophores: From Molecular Processes to Global Impact, eds. H. R. Thierstein and J. Young (Heidelberg: Springer, 75–97.
Tyrrell, T., Schneider, B., Charalampopoulou, A., and Riebesell, U. (2008). Coccolithophores and calcite saturation state in the Baltic and Black Seas. Biogeosciences 5, 485–494. doi: 10.5194/bg-5-485-2008
Tyrrell, T., and Zeebe, R. E. (2004). History of carbonate ion concentration over the last 100 million years. Geochim. Cosmochim. Acta 68, 3521–3530. doi: 10.1016/j.gca.,2004.02.018
United Nations Framework Convention on Climate Change (2015). Adoption of the Paris agreement: Proposal by the President to the United Nations Framework Convention on Climate Change. United Nations Framework Convention on Climate Change
van Vuuren, D. P., Stehfest, E., den Elzen, M. G. J., Kram, T., van Vliet, J., Deetman, S., et al. (2011). RCP2.6: exploring the possibility to keep global mean temperature increase below 2°C. Clim. Change 109, 95–116. doi: 10.1007/s10584-011-0152-3
Waldbusser, G. G., Hales, B., and Haley, B. A. (2015). Calcium carbonate saturation state: on myths and this or that stories. ICES J. Mar. Sci. 73, 563–568. doi: 10.1093/icesjms/fsv174
Walsh, M. R. (2013). The evolutionary consequences of indirect effects. Trends Ecol. Evol. 28, 23–29. doi: 10.1016/j.tree.2012.08.006
Webb, A. L., Malin, G., Hopkins, F. E., Ho, K.-L., Riebesell, U., Schulz, K., et al. (2016). Ocean acidification affects production of DMS and DMSP measured in a mesocosm study and cultures of Emiliania huxleyi RCC1229: a comparison of community interactions and laboratory monocultures. Environ. Chem. 13, 314–329. doi: 10.1071/EN14268
Wilken, S., Hoffmann, B., Hersch, N., Kirchgessner, N., Dieluweit, S., Rubner, W., et al. (2011). Diatom frustules show increased mechanical strength and altered valve morphology under iron limitation. Limnol. Oceanogr. 56, 1399–1410. doi: 10.4319/lo.2011.56.4.1399
Williamson, P. (2016). Emissions reduction: scrutinize CO2 removal methods. Nature 530, 153–155. doi: 10.1038/530153a
Wilson, J. D., Barker, S., and Ridgwell, A. (2012). Assessment of the spatial variability in particulate organic matter and mineral sinking fluxes in the ocean interior: implications for the ballast hypothesis. Glob. Biogeochem. Cycles 26:GB4011. doi: 10.1029/2012GB004398
Wilson, W., Zhang, Q., and Rickaby, R. E. M. (2019). Susceptibility of algae to Cr toxicity reveals contrasting metal management strategies. Limnol. Oceanogr. 9999, 1–12. doi: 10.1002/lno.11183
Wolf-Gladrow, D., and Riebesell, U. (1997). Diffusion and reactions in the vicinity of plankton: a refined model for inorganic carbon transport. Mar. Chem. 59, 17–34. doi: 10.1016/S0304-4203(97)00069-8
Wolf-Gladrow, D. A., Zeebe, R. E., Klaas, C., Körtzinger, A., and Dickson, A. G. (2007). Total alkalinity: the explicit conservative expression and its application to biogeochemical processes. Mar. Chem. 106, 287–300. doi: 10.1016/j.marchem.2007.01.006
Keywords: plankton, risk assessment, iron, nickel, silicon, silicification, calcification
Citation: Bach LT, Gill SJ, Rickaby REM, Gore S and Renforth P (2019) CO2 Removal With Enhanced Weathering and Ocean Alkalinity Enhancement: Potential Risks and Co-benefits for Marine Pelagic Ecosystems. Front. Clim. 1:7. doi: 10.3389/fclim.2019.00007
Received: 05 June 2019; Accepted: 13 September 2019;
Published: 11 October 2019.
Edited by:
Susan Davis Hovorka, University of Texas at Austin, United StatesReviewed by:
Lyla Taylor, University of Sheffield, United KingdomVolker Sick, University of Michigan, United States
Copyright © 2019 Bach, Gill, Rickaby, Gore and Renforth. This is an open-access article distributed under the terms of the Creative Commons Attribution License (CC BY). The use, distribution or reproduction in other forums is permitted, provided the original author(s) and the copyright owner(s) are credited and that the original publication in this journal is cited, in accordance with accepted academic practice. No use, distribution or reproduction is permitted which does not comply with these terms.
*Correspondence: Lennart T. Bach, bGVubmFydC5iYWNoQHV0YXMuZWR1LmF1